- 1Division of Toxicology, Leiden Academic Centre for Drug Research, Leiden University, Leiden, Netherlands
- 2Division of Immunology, Netherlands Cancer Institute, Amsterdam, Netherlands
In healthy individuals, immune-checkpoint molecules prevent autoimmune responses and limit immune cell-mediated tissue damage. Tumors frequently exploit these molecules to evade eradication by the immune system. Over the past years, immune-checkpoint blockade of cytotoxic T lymphocyte antigen-4 and programed death-1 emerged as promising strategies to activate antitumor cytotoxic T cell responses. Although complete regression and long-term survival is achieved in some patients, not all patients respond. This review describes promising, novel combination approaches involving immune-checkpoint blockade in the context of the cancer-immunity cycle, aimed at increasing response rates to the single treatments. Specifically, we discuss combinations that promote antigen release and presentation, that further amplify T cell activation, that inhibit trafficking of regulatory T cells or MSDCs, that stimulate intratumoral T cell infiltration, that increase cancer recognition by T cells, and that stimulate tumor killing.
Introduction
Historically, the importance of the immune system in restraining cancer development has been demonstrated by the observation that immune-deficient patients and organ-transplant recipients treated with immunosuppressive drugs have an increased risk to develop cancer (1). The steps involved in the development and maintenance of tumor immunity were recently conceptualized in the “cancer-immunity” cycle (and schematically depicted in Figure 1, outer circle) (2). This cycle describes the steps that are required to generate tumor-specific cytotoxic T lymphocytes [CTLs, also known as CD8+ (effector) T cells] that are capable of killing tumor cells. Defects/impediments in the cycle due to inhibitory receptor signaling frequently lead to evasion of tumor eradication by the immune system. This may explain the clinical success of immune-checkpoint therapies. Two important immune-checkpoint molecules include cytotoxic T lymphocyte antigen-4 (CTLA-4, also known as CD152) and programed death-1 (PD-1, also known as CD279).
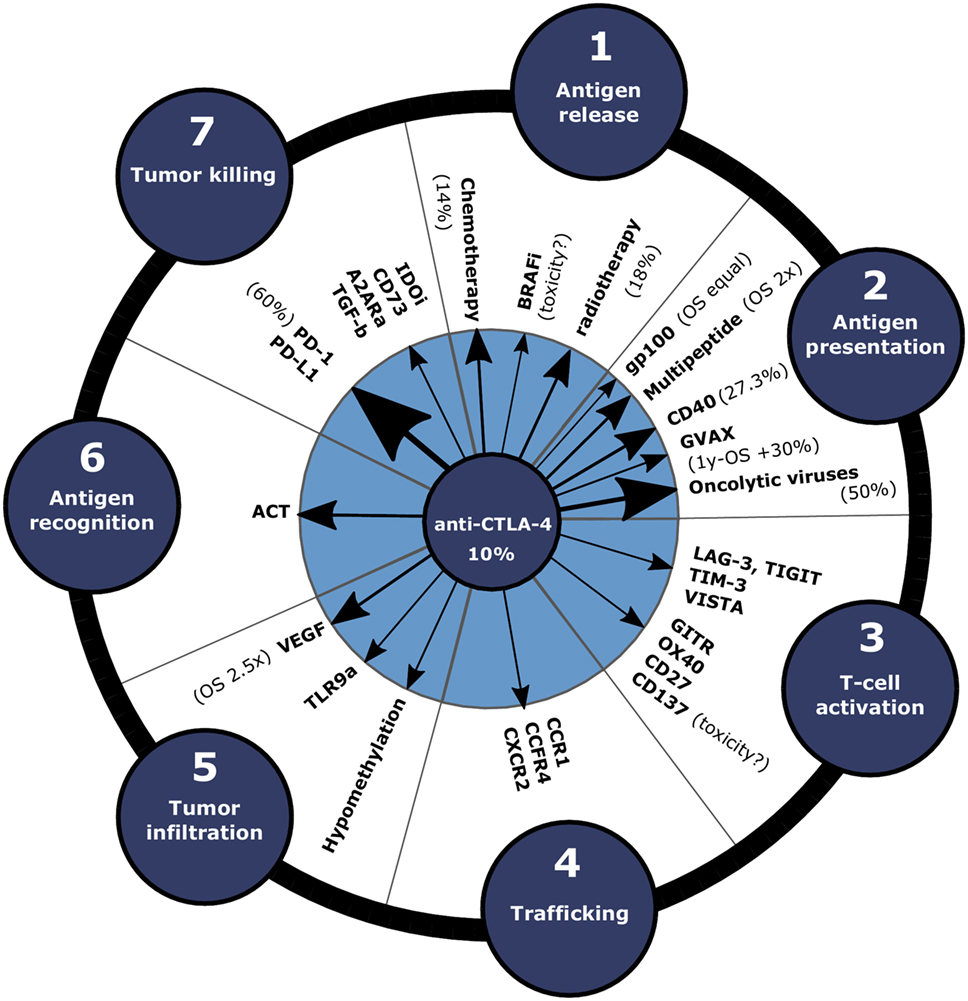
Figure 1. Clinical efficacy of combination approaches with anti-CTLA-4 depicted in the cancer-immunity cycle. In order to enhance the response rate of immune-checkpoint blockade, anti-CTLA-4 can be combined with therapies that promote various steps of the cancer-immunity cycle. Arrow size indicates the efficacy increase compared to anti-CTLA-4 monotherapy in clinical and preclinical studies. Clinical response rates or OS data compared to anti-CTLA-4 only for the treatment of melanoma patients are annotated between brackets. Abbreviations: A2ARa, adenosine 2a receptor antagonist; ACT, adoptive cell transfer; BRAFi, BRAFV600E inhibitors; GVAX, cellular vaccines consisting of irradiated GMCSF-producing tumor cells; IDOi, IDO inhibitor; MEKi, MEK inhibitors; OS, overall survival; TLR9a, TLR9 agonist.
Cytotoxic T Lymphocyte Antigen-4
Like CD28, CTLA-4 binds to the co-stimulatory molecules CD80 or CD86, but instead attenuates T cell activation (3). Therefore, CTLA-4 mainly exerts its immunosuppressive effect during activation in secondary lymphoid organs (Figure 2A) (4, 5). However, CTLA-4 signaling also enhances immunosuppression in other ways, e.g., by promoting the activity of regulatory T cells (Tregs) inside the tumor-microenvironment (6, 7), and the relative importance of these different mechanisms is not fully understood nor their contribution to therapy success. The anti-CTLA-4 monoclonal antibody ipilimumab (fully human IgG1, Bristol-Myers Squibb) increased the median overall survival (OS) among metastatic melanoma patients in a pooled analysis of phase II and III data and some patients even survived 10 years after treatment (8). However, 60% of the patients treated with anti-CTLA-4 experienced immune-related adverse events, which were severe (grade 3 or 4) in 10–15% of the patients (9).
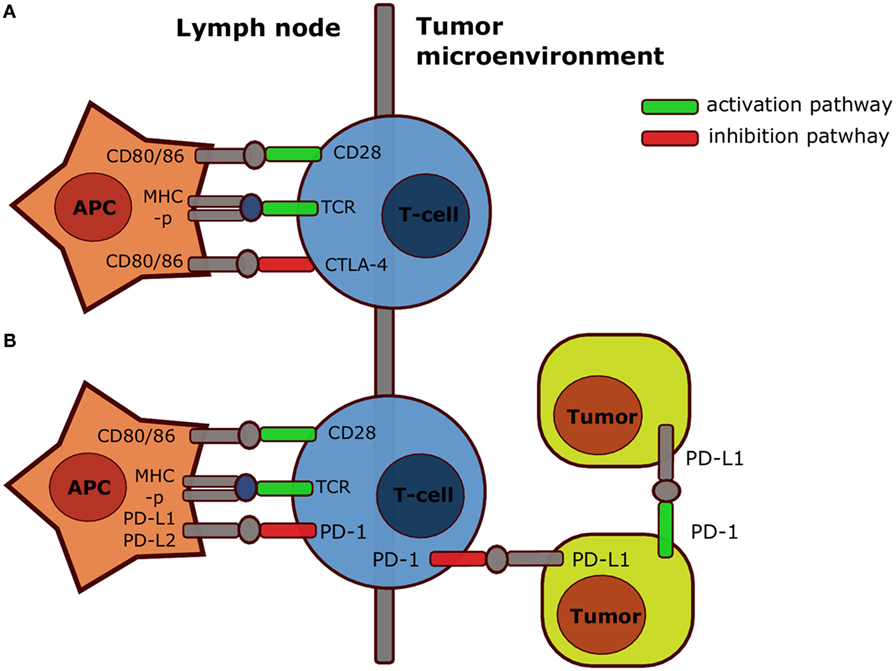
Figure 2. Simplified mechanism of action CTLA-4 and PD-1. Both CTLA-4 (A) and PD-1 (B) inhibit T cell activation in secondary organs after interaction with respectively B7 or PD-L1 and PD-L2. However, PD-1 also inhibits T cell responses after interaction with PD-L1 on tumor cells, and PD-1 expressed by a specific subset of tumor cells also contributes directly to tumorigenesis upon interaction with PD-L1 on tumor cells or stroma cells. Abbreviations: APC, antigen-presenting cell; CD, cluster of differentiation; CTLA-4, cytotoxic T lymphocyte antigen-4; MHC-p, peptide-major-histocompatibility complex; PD-1, programed death-1; PD-L1, programed death-ligand-1; TCR, T cell receptor. Note that the scheme is highly simplified: in reality CTLA-4 and PD-1 act through multiple mechanisms.
Anti-CTLA-4 treatment has also been studied in non-melanoma tumors, demonstrating an 8% partial response rate in patients with metastatic renal cell carcinoma (RCC) in a phase II study (10). Nevertheless, anti-CTLA-4 treatment is generally not effective in non-melanoma tumors, which might for instance be due to a low fraction of tumor-reactive T cells in these tumors, high expression of PD-L1, or the secretion of anti-inflammatory cytokines. Overall, long-term survival can be obtained with anti-CTLA-4 treatment in melanoma patients, but the toxicity is quite high and it is typically less effective in other tumor types.
Programed Death-1
Similar to CTLA-4, PD-1 signaling within secondary lymphoid organs impairs T cell priming and enhances differentiation into Tregs (11, 12). Moreover, because PD-L1 expression is frequently upregulated in cancers and tumor-infiltrating lymphocytes (TILs) can express high levels of PD-1 (4, 13), PD-1 inhibits T cell responses in the tumor. PD-1 can also be expressed on tumor cells, such as melanoma subpopulations, and contribute to tumorigenesis (14). Therefore, a part of the efficacy of anti-PD-1 therapy may be due to inhibition of this cell-intrinsic pathway active in some tumor cells (Figure 2B) (14). As expected from the predominantly peripheral role of PD-1, Pdcd1−/− (the gene encoding for PD-1) mice developed mild, organ-specific autoimmune responses (15, 16). Large phase I studies led to the prompt approval of the anti-PD-1 monoclonal antibodies pembrolizumab (humanized IgG4, Merck) and nivolumab (fully human IgG4, Bristol-Myers Squibb, Ono Pharmaceuticals) for patients with unresectable or metastatic melanoma not responding to anti-CTLA-4 (17–19). Importantly, anti-PD-1 was superior to anti-CTLA-4 in the treatment of advanced melanoma in terms of progression-free survival (PFS; 47.3 versus 26.5%) (20). Because severe (grade 3–5) side effects also occurred less frequently in anti-PD-1-treated (13.3%) compared with anti-CTLA-4-treated patients (19.9%), anti-PD-1 treatment is currently the first-line treatment for unresectable or metastatic melanoma in the USA and the EU. In addition, the FDA approved anti-PD-1 for the treatment of Hodgkin lymphoma, non-small-cell lung carcinoma (NSCLC), RCC, and head and neck squamous cell carcinoma (HNSCC), because clinical trials demonstrated the safety and efficacy in these cancer types (21–26). Anti-PD-1 might also improve the treatment of bladder, gastric, ovarian, and triple negative breast cancer (4, 19). Furthermore, anti-PD-L1 (atezolizumab) was recently approved for the treatment of bladder cancer (urothelial carcinoma) (27). In summary, anti-PD-1 is less toxic yet more effective than anti-CTLA-4 and is also effective in the treatment of non-melanoma tumors.
Improving Tumor Regression upon CTLA-4 or PD-1 Blockade
Despite the general success of checkpoint therapies, not all patients respond or achieve only partial tumor regression to anti-PD-1 or anti-CTLA-4 monotherapy (20). This is probably due to impediments somewhere in the cancer-immunity cycle (Figure 1): release of cancer antigens (step 1), antigen presentation (step 2), T cell priming and activation (step 3), T cell trafficking to tumors [step 4; note that, in this review, we specifically consider blocking the trafficking of immunosuppressive Tregs and myeloid-derived suppressor cells (MDSCs)], T cell infiltration into the tumor (step 5), cancer cell recognition by T cells (step 6), and killing of tumor cells (step 7). Therefore, higher response rates may be achieved using combination approaches of anti-PD-1 or anti-CTLA-4 with therapies that stimulate various steps of the cancer-immunity cycle, which we will discuss in this review. In brief, this involves combinations with conventional (e.g., chemotherapy and radiotherapy) and targeted therapies to promote antigen release (step 1) (28); combinations with vaccination to promote antigen presentation (step 2); combinations with agonists for co-stimulatory molecules or blockade of co-inhibitory molecules to further amplify T cell activation (step 3); combinations with trafficking inhibition of Tregs or MSDCs (step 4); combinations with anti-vascular endothelial growth factor (VEGF) to stimulate intratumoral T cell infiltration (step 5); combinations with adoptive cell transfer (ACT) to increase cancer recognition by T cells (step 6); and combinations that stimulate tumor killing (step 7). Finally, individualized treatment, based on biomarkers that predict clinical responses, could potentially optimize the management of various cancer types (29). In the following, we will discuss the progress with respect to the mentioned combination strategies step by step.
Combinations with Stimulation of Antigen Release and Danger Signals (Step 1)
Chemotherapy, targeted therapies, and radiotherapy can promote immunogenic cell death (ICD) of tumor cells. ICD results in the release of tumor antigens and “danger signals,” also known as damage-associated molecular patterns (DAMPS), such as calreticulin, ATP, type I IFN, and non-histone chromatin-binding protein high-mobility group box 1 (HMGB1) (30, 31). Binding to their receptors (CD91, the purinergic receptors P2RX7 and P2RY2, IFNAR, and the toll-like receptor TLR4, respectively) on DCs, results in their activation, enhanced antigen presentation, upregulation of co-stimulatory receptors, and induction of adaptive immune responses (32), whereas cell death that is “immunologically silent” induces tolerance.
Chemotherapy
Promising preclinical studies have shown that chemotherapy can indeed sensitize tumors to immune-checkpoint blockade by promoting T cell activation and infiltration into the tumor (33). Moreover, chemotherapy, such as by cisplatin, can also enhance responses to T cell based immune therapies by sensitizing the tumor cells to T cell-induced death rather than by ICD (34). For example, cisplatin has been shown to synergize with synthetic long peptide (SLP) vaccination and improve tumor-cell killing in a preclinical tumor model (35). With respect to the clinical application, chemotherapy (dacarbazine) combined with anti-CTLA-4 (ipilimumab) was first tested in metastatic melanoma patients. A phase II study showed that more patients responded to dacarbazine plus anti-CTLA-4 when compared to anti-CTLA-4 alone (14.3 versus 5.4%) (36). In addition, a phase III study demonstrated that this combination slightly increased the OS, when compared to dacarbazine alone (11.2 versus 9.1 months) (37) (note the difference between the latter two studies in terms of the monotherapy). However, immune-related severe adverse events (grade 3 or 4) also increased (56.3 versus 27.5%) (37).
Since chemotherapeutics promote antigen release and may promote the activation of tumor-specific T cells, combined chemotherapy and immune-checkpoint blockade may also be clinically effective in non-melanoma tumors. However, chemotherapy (paclitaxel and carboplatin) followed by anti-CTLA-4 (ipilimumab) resulted in only a minor increase of the immune-related PFS in a phase II and phase IIIb/IV study in NSCLC and extensive disease SCLC patients, compared with chemotherapy alone (38, 39). In conclusion, despite promising preclinical studies, combination therapies of immune-checkpoint blockade with chemotherapy have so far only slightly enhanced the clinical efficacy, while amplifying adverse effects. The lack of efficacy might be attributed to limited induction of ICD by chemotherapy. As ATP is thought to be important in the recruitment and activation of DCs during ICD, combinations of chemotherapy and inhibition of the ATP degrading enzyme CD39 followed by immune-checkpoint blockade might improve the clinical efficacy (40, 41).
Targeted Therapies
Targeted therapies can also restore T cell activation, but subsequent expression of PD-L1 on tumor cells can lead to immune escape. For example, clinical data demonstrated that such escape occurred within 10–14 days after BRAF inhibition in tumors of patients with metastatic melanoma (42). Thus, combination approaches with immune-checkpoint blockade may function synergistically. However, a phase I trial combining BRAFV600E inhibitor vemurafenib with anti-CTLA-4 (ipilimumab) in metastatic melanoma was halted due to severe hepatotoxicity (43). To date, such hepatoxity did not occur in an ongoing phase I trial with the BRAFV600E inhibitor dabrafenib in combination with anti-CTLA-4 (ipilimumab) (44, 45), but clinical efficacy data are not yet available. Because PD-L1 is upregulated on tumor cells following BRAF inhibition, and anti-PD-1 therapy is less toxic compared to anti-CTLA-4, combining BRAFV600E inhibitors with anti-PD-1 therapy seems promising. Indeed, preclinical data showed that the combination of anti-PD-1 or anti-PD-L1 with BRAF inhibition enhanced antitumor responses in a BRAF/PTEN inducible melanoma model (46), thus warranting clinical studies. Still, BRAFV600E inhibition can potentially lead to activation of MAPK signaling in T cells, since wild-type BRAF is not inhibited. This may result in exhaustion or enhance immune suppression (47).
Until recently, the preclinical and clinical assessment of MEK inhibitors (e.g., tramatinib) in combination with immune-checkpoint blockade was limited, because MEK inhibitors disrupt T cell function transiently in vitro (48). Nevertheless, a synergistic effect of trametinib plus anti-PD-1, anti-PD-L1, or anti-CTLA-4 was observed in the preclinical CT26 carcinoma model (49). Triple therapy with MEK inhibitors, BRAFV600E inhibitors, and anti-CTLA-4 may potentially optimize antitumor T cell responses with limited autoimmune side effects, since the additional MEK inhibitors might circumvent T cell hyper-activation caused by BRAF inhibition (47). Nevertheless, the combination of MEK inhibitors (trametinib), BRAFV600E inhibitors (dabrafenib), and anti-CTLA-4 (ipilimumab) resulted in severe gastrointestinal toxicity in patients with metastatic melanoma in a phase I/II study (45). In contrast, MEK inhibitors (trametinib) and BRAFV600E inhibitors (dabrafenib) have been successfully combined with anti-PD-L1 (durvalumab; MEDI4736) in melanoma patients in a phase I study (50). Thus, MEK or BRAFV600E inhibitors might be safely and effectively used in combination with anti-PD-L1, but little clinical efficacy data are currently available.
Radiotherapy
Radiotherapy induces local tumor cell death, but can also modulate local and systemic immunity, in essence acting as an in situ vaccine. Radiotherapy can promote CD8+ T cell responses to radiotherapy-induced peptides (51), natural (52, 53) or exogenous tumor antigens (54, 55), or by promoting immune control within the tumor-microenvironment (51, 56–58) [reviewed in Ref. (59)]. CD8+ T cells can contribute to radiotherapy-induced reduction of the primary tumor (55), and radiotherapy can even induce regression of lesions that reside outside the field of radiotherapy, called the “abscopal effect.” However, this is a very rare phenomenon, reported 34 times since 1969 [reviewed in Ref. (60)], indicating that radiotherapy alone sub-optimally engages the cancer-immunity cycle (or does not overrule all the impediments that may still be there).
However, local and systemic responses may be achieved at the same time when radiotherapy is combined rationally with immunotherapy. Indeed, the Demaria/Formenti groups first demonstrated that radiotherapy combined with anti-CTLA-4 improved control of irradiated tumors and reduced non-irradiated lung metastases in preclinical models (61, 62). Similarly, PD-L1 or PD-1 blockade and radiotherapy-induced local combined responses in mouse models for colon carcinoma (MC38) and mammary tumors (4T1 and TUBO) (63), and resulted in long-term survival in a mouse model for intracranial gliomas (GL261) (64). In the latter case, long-term immunologic memory was achieved, because rechallenged, long-term surviving mice did not develop new tumors.
A number of clinical case studies showed that concurrent treatment with radiotherapy and anti-CTLA-4 (ipilimumab) promoted an abscopal effect in metastatic melanoma (3 × 9.5 Gy) as well as in NSCLC (5 × 6 Gy in 10 days) (65, 66). However, it is unclear whether this effect is caused by the combination of radiotherapy and anti-CTLA-4 (systemic effect of combination) or anti-CTLA-4 alone (systemic effect of immunotherapy), since the latter control was obviously not included in these case studies.
Confirming the challenge of obtaining systemic combined effects between radiotherapy and aCTLA-4 comes from a phase I/II study with castration-resistant prostate cancer (CRPC) patients, in which the tumors were first irradiated (1 × 8 Gy), followed by anti-CTLA-4 (ipilimumab) administration 2 days later in an attempt to maximize antigen presentation (67). In this study, relatively few severe adverse events (10%) were reported and a complete response occurred. However, no differences in median survival were found in a phase III trial among metastatic CRPC patients applying radiotherapy plus anti-CTLA-4 (ipilimumab) or placebo (11.2 versus 10.0 months) (68), again indicating that systemic combined effects between radiotherapy and anti-CTLA-4 are sub-optimal. Finally, a recent phase I study in metastatic melanoma patients reported an 18% abscopal response rate, but this is also expected from anti-CTLA-4 treatment alone (69). Anti-CTLA-4 treatment can result in the upregulation of tumoral PD-L1 expression (69). Indeed, the addition of anti-PD-1 or anti-PD-L1 to radiotherapy plus anti-CTLA-4 in a mouse model increased local combined responses and achieved cures in up to 80% of the animals, but combined effects on non-irradiated tumors were not assessed in this setting (69).
Hence, high (local) response rates are currently only achieved in combination with double immune-checkpoint blockade, and convincing clinical abscopal effects with radiotherapy and immune-checkpoint blockade are yet to be reported. In order to optimize the efficacy of combination approaches with radiotherapy, it is important to mechanistically understand how radiotherapy can optimally induce ICD to kickstart a new T cell response, which likely depends on the tumor type and location (hypoxic versus normoxic environment) and how irradiated and distal tumor-microenvironment are influenced by radiotherapy. Then, irradiation dose, scheduling, size of radiotherapy field (note: want this as small as possible to prevent damage to circulating lymphocytes that circulate through the RT field), timing, and delivery method can be optimized to achieve local and systemic therapeutic effects with immune-checkpoint inhibitors (70–72). For more details on preclinical and clinical efficacy of radiotherapy combined with aCTLA-4 see an elegant, recent review by Vanpouille-Box et al. (73).
In summary, the preclinical studies on combination approaches of immune-checkpoint blockade with stimulation of antigen release are promising, yet the clinical efficacy is currently limited. Furthermore, combination therapies with chemotherapy of BRAF-targeted therapies are potentially accompanied by severe side effects. Combination approaches with radiotherapy seem to have a more favorable toxicity profile, but, to date, local and abscopal antitumor responses are limited.
Combinations with Stimulation of Antigen Presentation and Co-Stimulation (Step 2)
Peptide vaccines, live attenuated vaccines, cellular vaccines, oncolytic viruses, and agonists for co-stimulatory molecules on antigen-presenting cells (APCs), such as DCs, can be used to stimulate antigen presentation.
Peptide
In a phase I study with advanced melanoma patients, vaccination with a modified sequence from glycoprotein 100 (gp100), a melanoma tumor antigen, increased the frequency of melanoma-specific CD8+ T cells (74). Nevertheless, the tumors progressed, implying that vaccination may benefit from combination with immune-checkpoint blockade. However, anti-CTLA-4 (ipilimumab) plus gp100 peptide vaccine did not improve the OS, compared with anti-CTLA-4 alone (10.0 versus 10.1 months) in a phase III trial in advanced melanoma patients (9). Thus, multiple antigens may need to be targeted to induce sufficient antitumor CD8+ T cell responses. Such a multi-peptide vaccine (gp100, MART-1, NY-ESO-1) increased the number of gp100+, MART-1+, and NY-ESO-1+ CD8+ T cells in advanced melanoma patients when combined with anti-PD-1 (nivolumab), and a promising 1-year survival of 87% was obtained in this phase I trial (75). Thus, early clinical data indicate that multi-peptide vaccines combined with immune-checkpoint blockade are highly promising.
Live Attenuated Vaccines
Vaccination strategies in combination with immune-checkpoint blockade are also studied in non-melanoma tumors. Currently, a vaccine with a live-attenuated Listeria strain encoding the human papillomavirus (HPV) 16 oncoprotein E7 (ADXS11-001) in combination with anti-PD-L1 (durvalumab) is studied in a phase I/II trial in patients with cervical cancer or HPV-positive head and neck cancer (NCT02291055). Furthermore, a live-attenuated Listeria strain encoding for prostate-specific antigen level (PSA) (ADXS31-142) in combination with anti-PD-1 (pembrolizumab) is studied in a phase I/II trial in patients with prostate cancer (NCT02325557). In summary, combination approaches with live attenuated viruses are currently tested in the clinic, but clinical safety and efficacy data are not available yet.
Cellular Vaccines
Clinical trials combining checkpoint therapy with cellular vaccines are also ongoing, such as a phase I trial combining MART-126–35-loaded DC injection with anti-CTLA-4 (tremelimumab) in metastatic melanoma patients (76) and with anti-PD-1 (nivolumab) in patients with recurrent brain tumors (NCT02529072).
However, cellular vaccines consisting of irradiated GM-CSF-producing tumor cells (GVAX) may be more suitable, since they deliver multiple antigens. Granulocyte-macrophage colony-stimulating factor (GM-CSF) attracts DCs, stimulates the differentiation of monocytes into DCs, and amplifies DC cell maturation and antigen presentation (77, 78). Preclinical studies showed that subcutaneous injection of GVAX plus CTLA-4 blockade synergistically promoted tumor eradication in the B16 mouse melanoma model (response rate 80 versus 16% with GVAX alone) (79) due to an increase in CD8+ T cells and in effector T cell/Treg ratio in the tumor (80). GVAX plus PD-1 blockade also enhanced survival in the murine B16 melanoma, CT26 colon carcinoma, and pancreatic ductal adenocarcinoma models (81, 82). Furthermore, GVAX plus double checkpoint blockade (anti-CTLA-4 and anti-PD-1) enhanced tumor rejection to 100% in CT26 colon carcinoma and to 75% in ID8 ovarian carcinoma, compared to, respectively, 75 and 50% with double checkpoint blockade only (83). It should be noted that depigmentation was observed in 56% of the mice after combined GVAX and immune-checkpoint blockade (79), which correlated with antitumor activity. Clinical studies with cellular vaccines and checkpoint therapy are still rare. In a phase I/II trial with metastatic CRPC patients, treatment with GVAX plus anti-CTLA-4 (ipilimumab) was found to be safe and decreased the PSA by ≥50% in 25% of the patients (84). Thus, preclinical and early clinical data of cellular vaccines in combination with immune-checkpoint blockade are promising, warranting future clinical studies.
GM-CSF and Oncolytic Viruses
Granulocyte-macrophage colony-stimulating factor can also be directly administered instead of through production by irradiated tumor cells. In a phase II trial of systemic administration of recombinant GM-CSF (sargramostim) plus ipilimumab in metastatic melanoma patients, their 1-year survival increased upon combination therapy compared with anti-CTLA-4 alone (68.9 versus 52.9%) without toxicity differences (85). In addition, to minimize toxicity and optimize efficacy, GM-CSF can also be locally delivered in the tumor using modified oncolytic herpes viruses (talimogene laherperepvec; T-VEC) to produce GM-CSF. Such oncolytic viruses infect melanoma cells and then directly promote cell death with antigen release, stimulating antitumor immune responses (86). Intratumoral injection of T-VEC in melanoma patients in combination with ipilimumab treatment resulted in a response rate of 50% with a tolerable safety profile in a phase Ib trail. Currently, T-VEC combined with anti-CTLA-4 (ipilimumab) is studied in a phase II trial (NCT01740297) and combined with anti-PD-1 (pembrolizumab) in a phase Ib/III trail (NCT02263508), both in melanoma patients. The latter provided a clinical benefit and a phase III trial is planned (87, 88). Based on the clinical response rate of T-VEC and anti-CTLA-4, immune-checkpoint blockade with oncolytic viruses might be one of the most promising combination approaches.
Anti-CD40 and Anti-CD47
CD40 is constitutively expressed on APCs and, like GM-CSF, agonistic antibodies for CD40 (anti-CD40) provoke DC maturation and the expression of co-stimulatory molecules. A 27.3% response rate was obtained with anti-CD40 (CP-870893) plus anti-CTLA-4 (tremelimumab) in a phase I study in melanoma patients (NCT01103635) (89). As anti-CD40 has been shown to upregulate PD-L1 in a mouse model (90), higher response rates might be obtained with anti-CD40 plus anti-PD-1. Indeed, anti-CD40 (APX005M) is currently studied in combination with anti-PD-1 (pembrolizumab) in a phase I/II trial in melanoma patients (NCT02706353). Furthermore, combination therapies with antagonistic antibodies for CD47, which provide “do not eat me” signals to APCs, might be used in the future in order to stimulate the engulfment of antigens (91). Thus, combination approaches with anti-CD40 or anti-CD47 can also be used to improve antigen presentation, but the available data show a smaller effect than other approaches to affect antigen presentation.
Overall, both the preclinical and initial clinical data for combination therapy with immune-checkpoint blockade and multi-peptide vaccines and oncolytic viruses are promising, whereas the combination with single peptide vaccines and anti-CD40 so far seem less effective.
Combinations with Stimulation of T Cell Activation (Step 3)
Combination approaches of anti-CTLA-4 or anti-PD-1 with the blockade of other immune-checkpoints or with activation of co-stimulatory molecules may also further amplify antitumor immune responses.
Double Immune-Checkpoint Blockade
CTLA-4 plus PD-1 Blockade
Preclinical models revealed that blocking of CTLA-4 or PD-1 alone led to upregulation of the unblocked pathway (92); hence, the efficacy of either monotherapy is limited by increased suppression of T cell responses through the other of the two pathways. Indeed, combined blockade of CTLA-4 and PD-1 prolonged survival in a B16 melanoma model (92), and combined PD-L1 and CTLA-4 blockade prolonged disease-free survival in the K7M2 model for metastatic osteosarcoma (93). This was due to enhanced effector T cell infiltration, proliferation, inflammatory cytokine production (e.g., IFN-γ), and an increase in the ratio of CD8+ T cells to Tregs and MDSCs in the tumor (92, 94).
Phase II clinical trials involving combination treatment with anti-CTLA-4 (ipilimumab) and anti-PD-1 (nivolumab) resulted in a higher response rate (61 versus 11% with anti-CTLA-4 only), more complete responses (22 versus 0% with anti-CTLA-4 only), and higher PFS in a phase III trial (11.2; anti-CTLA-4 2.9; anti-PD-1 6.9 months) (95, 96). However, severe (grade 3 or 4) treatment-related autoimmune adverse events were also amplified (55%; anti-CTLA-4 27.3%; anti-PD-1 16.3%) (96). In 2015, these studies led to the accelerated approval of anti-CTLA-4 (ipilimumab) in combination with anti-PD-1 (nivolumab) for the treatment of unresectable or metastatic melanoma in the USA. Still, optimization of this combination therapy, other combination approaches, or an individualized treatment is required to obtain clinical responses in a fraction of the patients above the current 60%. Currently, a phase I/II trial combining anti-CTLA-4 and anti-PD-1 therapy is ongoing in patients with solid tumors or sarcomas (NCT02304458), which will provide information about the efficacy and safety of double immune-checkpoint blockade in tumor types other than melanoma. Overall, double immune-checkpoint blockade with anti-CTLA-4 anti-PD-1 is currently the most effective combination approach and is approved for the treatment of melanoma.
Novel Immune Checkpoints
The immune-checkpoint molecules lymphocyte-activation gene-3 (LAG-3), T cell ITIM domain (TIGIT), or T cell immunoglobulin and mucin domain-3 (TIM-3) may also contribute to the immune evasion of tumor cells because they are primarily expressed on exhausted T cells (97–100). Various combinations have been tested in mouse models (Table 1). Blocking PD-1 and LAG-3 is particularly interesting because both are mainly expressed on TILs (101), which may result in a favorable safety profile due to restriction of T cell responses to the tumor-microenvironment. Results for this combination were promising in fibrosarcoma and colon cancer models, but tumor growth in the B16 melanoma model was not affected (101), possibly due to low LAG-3 and PD-1 expression on TILs in the B16 model. These observations highlight the importance to establish biomarkers that predict treatment responses to these combination approaches.
Furthermore, the inhibitory ligand V-domain immunoglobulin (Ig)-containing suppressor of T cell activation [VISTA, also known as PD-1 homolog (PD-1H)] is expressed on hematopoietic cells (e.g., T cells) and in particular on myeloid cells in tumors (108–110). The combination of anti-VISTA and anti-PD-1 synergistically promoted regression and long-term survival in a colon carcinoma (CT26) model (106). Therefore, it would be interesting to test this combination in the clinic.
In addition to T cell immune checkpoints, NK cells express killer-cell immunoglobulin-like receptors (KIRs), which inhibit the cytotoxic activity of NK cells after interaction with MHC-I on tumor cells (111). Co-blockade of PD-1 or CTLA-4 and KIR might be beneficial due to the activation of both T- and NK cells. Currently, the effect of combination therapy with anti-KIR (lirilumab) and anti-PD-1 (nivolumab; NCT01714739) or anti-CTLA-4 (ipilimumab; NCT01750580) are investigated in phase I clinical studies in patients with advanced solid tumors. In summary, combination therapies with novel immune checkpoints might have a more favorable safety profile compared to anti-CTLA-4 with anti-PD-1, and clinical assessment of these approaches is needed.
Co-Stimulatory Molecules
Like immune-checkpoint blockade, agonistic antibodies for co-stimulatory molecules (e.g., CD27, CD137, GITR, and OX40) amplify T cell activation and, as a consequence, they may also enhance antitumor T cell responses. Various combinations with checkpoint blockade have been tested in mouse models (Table 2). The combination of OX40 and anti-CTLA-4 is synergistic in ovarian carcinoma (ID8), fibrosarcoma (MCA-205), and prostate cancer (TRAMP1) models (112, 113). Moreover, anti-GITR and anti-PD-1 have synergistic effects in the ID8 model (114). Some of these combinations have potentially less autoimmune side effects than double immune-checkpoint blockade while still having beneficial effects, for example, combination therapy involving anti-CD137 and CTLA-4 blockade in MC38 colon carcinoma tumors and GL261 glioblastoma (115, 116). In contrast, this combination treatment had no effect on B16 melanoma tumors (115, 116), which may be due to increased PD-L1 expression on those tumors.
Since PD-L1 expression can confer resistance to anti-CD137 treatment (122), combined CD137 activation and PD-1 rather than CTLA-4 blockade may further improve antitumor responses. Indeed, anti-CD137 plus anti-PD-1 resulted in increased efficacy in mouse models for colon carcinoma (ID8), melanoma (B16F10), and ovarian carcinoma (ID8) (117, 120, 121). Furthermore, triple therapy (activation of CD137 and blockade of PD-1 and CTLA-4) was even more effective in the ID8 model (117). However, CD137 agonists have been reported to induce severe liver inflammation (123, 124), and, therefore, more studies determining the toxic effects of combinations involving such agonists are needed. Moreover, intratumoral administration may help to minimize liver toxicity. Although liver inflammation was not detected in combination with anti-CTLA-4 or anti-PD-1 in preclinical studies (115, 117, 120), caution should be taken during its clinical assessment. Currently, a phase I study, in which the toxicity of anti-CD137 and anti-PD-1 is determined in patients with solid tumors, is in progress (NCT02179918). Combination approaches with anti-CD27 (Tnfrsf7) are also promising (125). The combination of anti-CD27 with anti-PD-1, but not anti-CTLA-4, eradicated tumors in a cervical cancer model (TC-1) (119). Currently, the combination of anti-CD27 (varlilumab) with anti-CTLA-4 (ipilimumab) or anti-PD-1 (nivolumab) is investigated in respectively patients with melanoma (NCT02413827) and solid tumors (NCT02335918) in phase I/II studies. Like the combination approaches with blockade of novel immune checkpoints, agonists for co-stimulatory molecules might have a more favorable safety profile, but caution is required with anti-CD137.
Summarizing the combinations with T cell stimulation, double immune-checkpoint blockade of CTLA-4 and PD-1 dramatically increased the response rate compared with monotherapy, but personalized treatment of anti-PD-1 with new immune-checkpoint inhibitors or agonists for co-stimulatory molecules can potentially minimize the severe side effects observed with CTLA-4/PD-1 combined blockade.
Combinations with Trafficking Inhibition of Tregs or MSDCs (Step 4)
Apart from recruiting antitumor T cells in step 4 of the cancer-immunity cycle, tumors also attract MDSCs and Tregs, which contribute to evasion of immune destruction. Therefore, trafficking inhibition of MDSCs and Tregs to the tumor using specific chemokine receptor inhibitors could abrogate immune evasion and improve antitumor T cell responses. Subpopulations of MDSCs express high levels of C–C chemokine receptor 1 (CCR1) or CXC chemokine receptor 2 (CXCR2) (126, 127). Their ligands, respectively, CCL5 (MCP-1), CCL7 (RANTES), and CXCL8, are secreted by tumors and mediate the recruitment of CCR1 or CXCR2 positive MDSCs. Combination of a CCR1 antagonist (CCX9588) and anti-PD-L1 synergistically reduced the tumor burden in a preclinical breast cancer model (126). Moreover, anti-CXCR2 plus anti-PD-1 enhanced survival in a rhabdomyosarcoma (RMS) model (127).
Regulatory T cells (FOXP3hi and CD45RA−) express high levels of CCR4 in the blood and inside tumors (128). Therefore, combination approaches of immune-checkpoint blockade and inhibition of Treg recruitment employing anti-CCR4 is promising. In addition, anti-CCR4 promotes antibody-dependent cell-mediated cytotoxicity (ADCC), which may further reduce the Treg population (129). Currently, anti-CCR4 (mogamulizumab) in combination with anti-PD-1 (nivolumab; NCT02705105, phase I/II), anti-PD-L1 (durvalumab; NCT02301130, phase I), and anti-CTLA-4 (tremelimumab; NCT02301130, phase I) is being tested in the clinic in patients with various advanced solid tumors. In summary, preclinical studies indicate the potential of trafficking inhibition of Tregs or MDSCs, but clinical efficacy data are not reported yet.
Combinations with T Cell Infiltration Stimulators (Step 5)
Deficient T cell infiltration can contribute to tumor immune escape because of the absence of sufficient numbers of T cells. T cell infiltration can be stimulated using anti-VEGF, agonists for innate immune receptors, and epigenetic modification.
Vascular Endothelial Growth Factor
The growth factor VEGF inhibits T cell infiltration into the tumor by downregulation of adhesion molecules on endothelial cells (130). However, the immunosuppressive function of VEGF is much more diverse. For instance, VEGF also inhibits antigen presentation by DCs, enhances the expansion of Tregs, and mediates PD-1 upregulation on tumor-infiltrated T cells (131–133). High VEGF levels are associated with decreased OS in advanced melanoma patients after treatment with anti-CTLA-4 (134). Therefore, the combination of anti-VEGF-A (bevacizumab; humanized IgG1, Roche) and anti-CTLA-4 (ipilimumab) was investigated in a phase I trial in advanced melanoma patients. This combination resulted in a high CD8+ T cell infiltration and a median survival of 25.1 months (135), suggesting a substantial benefit compared to the 10.1 months median survival of anti-CTLA4 alone (9) (note though that this is another trial). In this trial, 28.3% of the patients experienced severe (grade 3 or 4) adverse events (135).
Another phase I trial applying a small molecule tyrosine kinase VEGF receptor (VEGFR) inhibitor (sunitinib) plus anti-CTLA-4 (tremelimumab) to patients with metastatic RCC resulted in acute renal failure, and the study was therefore halted (136). However, combined treatment with the generally safer anti-PD-1 and the VEGFR inhibitor sunitinib may be feasible. In preclinical studies, the combination of anti-VEGF-A and anti-PD-1 therapy was more effective than the monotherapies in a CT26 carcinoma mouse model (131). Moreover, preliminary clinical data of a phase I trial investigating the combination of anti-PD-1 (nivolumab) with either the VEGFR inhibitor sunitinib or with a multikinase VEGFR inhibitor (pazopanib) are promising (137, 138). In summary, combination therapies with anti-VEGF might provide a substantial survival benefit to patients, whereas caution is required with VEGFR inhibitor combination therapies due to renal toxicity.
Innate Immune Receptors
Innate immune receptors (also known as pattern recognition receptors; PRR) such as TLR can detect a broad range of pathogen- and danger-associated patterns. Binding of these ligands to TLRs on DCs results in their maturation (139, 140). In addition, TLR9 agonists have been shown to increase T cell infiltration in the CT26 colon cancer model (141). Therefore, TLR9 agonists might be used to enhance T cell infiltration into the tumor. Currently, anti-CTLA-4 (ipilimumab) combined with a TLR9 agonist (MGN1703) is studied in a phase I trial in patients with advanced solid malignancies (NCT02668770). In summary, although clinical data are not yet available, combinations with innate immune receptor stimulation might improve T cell infiltration.
Epigenetic Modulation
Epigenetic silencing of immune-related genes using hypermethylation contributes to immune evasion during tumor progression (142, 143). Hypomethylating agents, of which the nucleoside DNA methylation inhibitor Azacitidine (AZA) and 5-aza-2′-deoxycitidine (5AZA2) are most extensively studied, can be used to restore gene expression (144). 5AZA2 treatment has been shown to promote CD8+ T cell infiltration into the tumor in the EL4 lymphoma model, which was attributed to demethylation-induced CD80 expression on tumor cells (145). Therefore, hypomethylation can be used as a strategy to stimulate T cell infiltration into the tumor. Moreover, 5AZA2 treatment combined with anti-CTLA-4 is synergistic in murine mammary carcinoma TS/A and in mesothelioma AB1 models and results in high CD8+ and CD4+ T cell infiltration (146). Thus, clinical studies are needed as a next step to investigate the safety and efficacy of combination approaches with epigenetic modulation.
In conclusion, several approaches to stimulate T cell infiltration have been attempted but our knowledge on the efficacy of T cell infiltration stimulators combined with checkpoint blockade remains limited. This clearly is an area where more research is needed.
Combinations with Antigen Recognition Stimulators (Step 6)
Adoptive cell transfer is another promising strategy for the treatment of melanoma with objective response rates of approximately 50% and complete tumor regression in 22% of the patients (147). This approach involves the ex vivo culture of a tumor biopsy, the expansion and selection of TILs for tumor specificity, and reinfusion of the tumor-reactive cells into the patient. Instead of TILs, T cells expressing antigen-specific chimeric antigen receptors (CARs) are alternatively used to target specific tumor antigens (148). However, the lack of sufficient T cell infiltration into the tumor, as well as an immunosuppressive tumor-microenvironment may limit ACT success in some patients (149). Indeed, ACT plus anti-PD-1, anti-PD-L1, or anti-CTLA4 synergistically reduced tumor growth in the MC38 carcinoma, B16 and B16F10 melanoma mouse models (150–152) and increased the long-term survival in transgenic Her-2 mice upon ACT of Her-2+-specific CAR T cells and anti-PD-1 compared to the monotherapies (153). Combination therapy enhanced the proliferation of T cells within tumors, their cytotoxic activity, and IFN-γ production, which mediated chemokine upregulation (e.g., CXCL10) and further T cell infiltration (150). Thus, the application of immune-checkpoint blockade after ACT may result in complete tumor regression in a large population in the clinic. Currently, the combination of ACT and anti-CTLA-4 is studied in a phase II study in patients with metastatic melanoma (NCT02027935).
Because anti-CTLA-4 and anti-PD-1 promote intratumoral T cell infiltration, immune-checkpoint blockade prior to ACT may increase the number of TILs that can be derived from a tumor biopsy. Moreover, expanded TILs derived from anti-CTLA4-treated patients have a less exhausted phenotype, which is associated with improved clinical responses (154). In summary, applying immune-checkpoint blockade either before or after ACT is a promising approach, but clinical data are currently lacking.
Combinations That Increase Tumor Killing (Step 7)
As discussed, tumor cells can evade killing via PD-L1 expression, since the interaction of PD-L1 with PD-1 inhibits the secretion of cytotoxic mediators by CD8+ T cells (2). Moreover, other molecules expressed on APCs or tumor cells may limit killing, such as indoleamine 2,3-dioxygenase (IDO), or immune suppressive mediators accumulating in the tumor environment, like adenosine and transforming growth factor β (TGF-β). Note that also the earlier discussed approaches of chemo- and radiotherapy can help to sensitize tumor cells to T cell-induced death.
Adenosine
Adenosine inhibits T cell proliferation and cytotoxicity upon binding to the A2A receptor on T cells (155–157). In addition, adenosine can directly provoke metastasis upon interaction with the A2B receptor on tumor cells (158). As a consequence, the combination of an A2A receptor antagonist and anti-CTLA-4 or anti-PD-1 synergistically inhibited (metastatic) tumor growth in breast cancer (4T1) and melanoma (B16F10) preclinical models (159–161). Currently, the safety and tolerability of combined A2A receptor antagonist (CPI-444) and anti-PD-L1 (atezolizumab) treatment is studied in a phase I trial in advanced cancers (NCT02655822).
Adenosine is formed by dephosphorylation of adenosine monophosphate (AMP) by the ecto-enzyme CD73 (162). Thus, CD73 has an immunosuppressive and pro-metastatic effect. Moreover, CD73 stimulates angiogenesis through upregulated VEGF secretion by tumor cells (163). As a consequence, high expression of the enzyme CD73 is associated with a poor prognosis in various cancer types (164–166). This enzyme is also a potential biomarker for anti-PD-1 therapy, since CD73 expression limits anti-PD-1 efficacy (161, 167). Indeed, both combined treatment with anti-CD73 and anti-CTLA-4 or anti-PD-1 enhanced the antitumor activity in colon (MC38), prostate (RM-1), and metastatic breast cancer (4T1.2) models (168). Currently, the combination of anti-CD73 (MEDI9447) plus anti-PD-L1 (durvalumab) is investigated in the clinic in patients with advanced solid cancer (NCT02503774) in a phase I study. In summary, combination approaches with A2A receptor antagonists and anti-CD73 to improve tumor killing are currently studied in the clinic due to their success in preclinical studies.
Transforming Growth Factor-β
Transforming growth factor-β can also contribute to immune suppression by stimulating Tregs (169). Preclinically, combined treatment with a TFG-β receptor kinase inhibitor I and anti-CTLA-4 synergistically inhibited primary and metastatic tumor growth in a melanoma model (BRAFV600EPTEN−/−) (170). The combination of the TFG-β receptor kinase inhibitor I (galunisertib) and anti-PD-L1 (durvalumab) or anti-PD-1 (nivolumab) are currently being clinically tested in patients with metastatic pancreatic cancer (NCT02734160, phase I) and NSCLC, hepatocellular carcinoma, or glioblastoma (NCT02423343, phase I/II). These clinical studies will demonstrate if combination approaches with TGF-β receptor kinase inhibitors may improve the treatment of various tumors.
In addition, TGF-β may impair radiotherapy-induced T cell priming, and TGF-β blocking antibodies can enhance T cell priming (step 2–3) and promote abscopal responses induced by (fractionated) radiotherapy in the 4T1 mouse model (53).
IDO
The presence of the tryptophan degrading enzyme IDO on APCs or tumor cells may also limit the killing efficacy of CTLA-4 or PD-1 blockade because tryptophan is required for T cell proliferation (171). Indeed, several preclinical studies demonstrated a survival benefit of combinations of anti-CTLA-4 or anti-PD-L1 with IDO inhibition by 1-methyl-d-tryptophan (1MT) treatment (Table 1). Thus, combination approaches with IDO inhibition may be efficacious, especially in patients with a high IDO expression. Finally, triple immune-checkpoint blockade of CTLA-4, PD-1, and IDO could further improve antitumor responses, but this approach probably does not increase the effectiveness compared to double checkpoint blockade in more established tumors (107).
Early clinical safety and efficacy data for the IDO1 inhibitor (epacadostat) in combination with anti-PD-1 (pembrolizumab) were promising for the treatment of various advanced cancers in a phase I/II study (e.g., melanoma, RCC, and NSCLC) (172). Currently, the IDO inhibitor epacadostat is also being tested in combination with anti-PD-1 (nivolumab; NCT02327078) and anti-PD-L1 (durvalumab; NCT02318277) for various cancers in phase I/II studies. In addition, 1MT (indoximod) plus anti-CTLA-4 (ipilimumab) or anti-PD-1 is being tested in melanoma patients (NCT02073123) in a phase I/II study. Thus, both preclinical and early clinical studies involving IDO inhibition have yielded promising results. However, because IDO inhibition seems particularly effective in tumors with a high IDO expression, it would be useful to target these patients specifically.
Summarizing the approaches that stimulate killing by T cells, various clinical trials currently investigate combination therapies that enhance such killing, but to date clinical safety and efficacy data are limited.
Conclusion
Various combination approaches with immune-checkpoint blockade have been studied in preclinical models, but to date the clinical efficacy and toxicity data are limited. It is difficult to compare the efficacy of the preclinical studies, since various cell lines and tumor models are used. A step forward here would be to employ a combination of experimental approaches and mathematical modeling to quantify the CTL-mediated killing rates (173, 174) for tumor destruction in different settings (i.e., tumor models, treatments) as well as the variability of these rates among mice, taking into account factors such as T cell infiltration and tumor size.
Double immune-checkpoint blockade or combinations with agonists for co-stimulatory molecules are effective at amplifying T cell activation. High response rates have especially been obtained by the combination of CTLA-4 and PD-1 and as a consequence this combination was approved in 2015 for the treatment of melanoma. Nevertheless, these approaches are not effective in all patients. Preclinical data suggest that complete tumor regression may be achieved in a large part of the population after combined treatment with ACT and immune-checkpoint blockade, but its widespread employment may be limited due to extensive laboratory requirements and the high costs involved. Initial clinical data also indicate that combination approaches with multi-peptide vaccines are effective, but patients with non-melanoma tumors expressing low levels of tumor antigens do not benefit from such an approach. In order to improve the efficacy of immune-checkpoint blockade in patients with non-melanoma tumors, combinations with chemotherapy or targeted therapies may be effective, since they induce antigen release and provide danger signals, but the adverse effects are often severe. Combined double immune-checkpoint blockade and radiotherapy might be a promising combination therapy in case the abscopal responses can be optimized (Figure 1). Moreover, the optimal timing of immune-checkpoint blockade relative to the “backbone” radiotherapy needs to be determined. Although combination approaches can increase the fraction of patients that respond to treatment in various cancer types, individualized immune-checkpoint combination approaches, based on predictive biomarkers, has an even higher potential to ensure that each patient is provided with the optimal care. Therefore, establishing biomarkers specifically for combination therapies involving immune-checkpoint blockade has high priority in future research.
Author Contributions
MS and JB conceptualized the manuscript; MS drafted the manuscript and composed the figures; and MS, JB, and IV carefully revised the manuscript. All the authors read and approved the final manuscript.
Conflict of Interest Statement
The authors declare that the research was conducted in the absence of any commercial or financial relationships that could be construed as a potential conflict of interest.
Funding
This work was supported by a Bas Mulder award from the Dutch Cancer Society/Alpe d’Huzes (NKI2013-5951, to IV) and by a Vidi grant from NWO (864.12.013, to JB).
Abbreviations
1MT, 1-methyl-d-tryptophan; ACT, adoptive cell transfer; APC, antigen-presenting cell; BRAFi, BRAF inhibitor; CARs, chimeric antigen receptors; CD, cluster of differentiation; CR, complete response; CRPC, castration-resistant prostate cancer; CTL, cytotoxic T lymphocyte; CTLA-4, cytotoxic T lymphocyte antigen-4; CXCL, C–X–C motif chemokine ligand; GITR, glucocorticoid-induced TNFR family-related gene; GM-CSF, granulocyte-macrophage colony-stimulating factor; gp100, glycoprotein 100; GVAX, cellular vaccines consisting of irradiated GM-CSF producing tumor cells; HNSCC, head and neck squamous cell carcinoma; HPV, human papillomavirus; ICD, immunogenic cell death; IDO, indoleamine 2,3-dioxygenase; IFN-γ, interferon gamma; KIRs, killer-cell immunoglobulin-like receptors; LAG-3, lymphocyte-activation gene-3; MAPK, mitogen-activated protein kinases; MART-1, protein melan-A; MEKi, mitogen-activated protein kinase kinase inhibitor; MHC, major histocompatibility complex; NCT, national clinical trial; NK cell, natural killer cell; NSCLC, non-small-cell lung carcinoma; NY-ESO-1, cancer-testis antigen; ORR, overall response rate; OS, overall survival; PD-1, programed death-1; PD-L1,2, programed death-ligand 1,2; PFS, progression-free survival; PSA, prostate-specific antigen; PTEN, phosphatase and tensin homolog; RCC, renal cell carcinoma; SLP, synthetic long peptide; TCR, T cell receptor; TG, tumor growth; TIGIT, T cell ITIM domain; TIL, tumor-infiltrating lymphocyte; TIM-3, T cell immunoglobulin and mucin domain-3; TNFR, tumor necrosis factor receptor; Treg, regulatory T cell; TV, tumor volume; VEGF, vascular endothelial growth factor; VEGFR, VEGF receptor.
References
1. Corthay A. Does the immune system naturally protect against cancer? Front Immunol (2014) 5:197. doi:10.3389/fimmu.2014.00197
2. Chen DS, Mellman I. Oncology meets immunology: the cancer-immunity cycle. Immunity (2013) 39(1):1–10. doi:10.1016/j.immuni.2013.07.012
3. Pardoll DM. The blockade of immune checkpoints in cancer immunotherapy. Nat Rev Cancer (2012) 12(4):252–64. doi:10.1038/nrc3239
4. Topalian SL, Drake CG, Pardoll DM. Immune checkpoint blockade: a common denominator approach to cancer therapy. Cancer Cell (2015) 27(4):450–61. doi:10.1016/j.ccell.2015.03.001
5. Kvistborg P, Philips D, Kelderman S, Hageman L, Ottensmeier C, Joseph-Pietras D, et al. Anti-CTLA-4 therapy broadens the melanoma-reactive CD8+ T cell response. Sci Transl Med (2014) 6(254):254ra128. doi:10.1126/scitranslmed.3008918
6. Peggs KS, Quezada SA, Chambers CA, Korman AJ, Allison JP. Blockade of CTLA-4 on both effector and regulatory T cell compartments contributes to the antitumor activity of anti-CTLA-4 antibodies. J Exp Med (2009) 206(8):1717–25. doi:10.1084/jem.20082492
7. Blank CU, Enk A. Therapeutic use of anti-CTLA-4 antibodies. Int Immunol (2015) 27(1):3–10. doi:10.1093/intimm/dxu076
8. Schadendorf D, Hodi FS, Robert C, Weber JS, Margolin K, Hamid O, et al. Pooled analysis of long-term survival data from phase II and phase III trials of ipilimumab in unresectable or metastatic melanoma. J Clin Oncol (2015) 33(17):1889–94. doi:10.1200/JCO.2014.56.2736
9. Hodi FS, O’Day SJ, McDermott DF, Weber RW, Sosman JA, Haanen JB, et al. Improved survival with ipilimumab in patients with metastatic melanoma. N Engl J Med (2010) 363(8):711–23. doi:10.1056/NEJMoa1003466
10. Yang JC, Hughes M, Kammula U, Royal R, Sherry RM, Topalian SL, et al. Ipilimumab (anti-CTLA4 antibody) causes regression of metastatic renal cell cancer associated with enteritis and hypophysitis. J Immunother (2007) 30(8):825–30. doi:10.1097/CJI.0b013e318156e47e
11. Goldberg MV, Maris CH, Hipkiss EL, Flies AS, Zhen L, Tuder RM, et al. Role of PD-1 and its ligand, B7-H1, in early fate decisions of CD8 T cells. Blood (2007) 110(1):186–92. doi:10.1182/blood-2006-12-062422
12. Francisco LM, Salinas VH, Brown KE, Vanguri VK, Freeman GJ, Kuchroo VK, et al. PD-L1 regulates the development, maintenance, and function of induced regulatory T cells. J Exp Med (2009) 206(13):3015–29. doi:10.1084/jem.20090847
13. Okazaki T, Honjo T. PD-1 and PD-1 ligands: from discovery to clinical application. Int Immunol (2007) 19(7):813–24. doi:10.1093/intimm/dxm057
14. Kleffel S, Posch C, Barthel SR, Mueller H, Schlapbach C, Guenova E, et al. Melanoma cell-intrinsic PD-1 receptor functions promote tumor growth. Cell (2015) 162(6):1242–56. doi:10.1016/j.cell.2015.08.052
15. Nishimura H, Nose M, Hiai H, Minato N, Honjo T. Development of lupus-like autoimmune diseases by disruption of the PD-1 gene encoding an ITIM motif-carrying immunoreceptor. Immunity (1999) 11(2):141–51. doi:10.1016/S1074-7613(00)80089-8
16. Nishimura H, Okazaki T, Tanaka Y, Nakatani K, Hara M, Matsumori A, et al. Autoimmune dilated cardiomyopathy in PD-1 receptor-deficient mice. Science (2001) 291(5502):319–22. doi:10.1126/science.291.5502.319
17. Robert C, Ribas A, Wolchok JD, Hodi FS, Hamid O, Kefford R, et al. Anti-programmed-death-receptor-1 treatment with pembrolizumab in ipilimumab-refractory advanced melanoma: a randomised dose-comparison cohort of a phase 1 trial. Lancet (2014) 384(9948):1109–17. doi:10.1016/S0140-6736(14)60958-2
18. Larkin J, Lao CD, Urba WJ, McDermott DF, Horak C, Jiang J, et al. Efficacy and safety of nivolumab in patients with BRAF V600 mutant and BRAF wild-type advanced melanoma. JAMA Oncol (2015) 1(4):433. doi:10.1001/jamaoncol.2015.1184
19. Ascierto PA, Marincola FM. 2015: the year of anti-PD-1/PD-L1s against melanoma and beyond. EBioMedicine (2015) 2(2):92–3. doi:10.1016/j.ebiom.2015.01.011
20. Robert C, Schachter J, Long GV, Arance A, Grob JJ, Mortier L, et al. Pembrolizumab versus ipilimumab in advanced melanoma. N Engl J Med (2015) 372(26):150419053123009. doi:10.1056/NEJMoa1503093
21. Borghaei H, Paz-Ares L, Horn L, Spigel DR, Steins M, Ready NE, et al. Nivolumab versus docetaxel in advanced nonsquamous non–small-cell lung cancer. N Engl J Med (2015) 373(17):1627–39. doi:10.1056/NEJMoa1507643
22. Garon EB, Rizvi NA, Hui R, Leighl N, Balmanoukian AS, Eder JP, et al. Pembrolizumab for the treatment of non–small-cell lung cancer. N Engl J Med (2015) 372(21):2018–28. doi:10.1056/NEJMoa1501824
23. Brahmer J, Reckamp KL, Baas P, Crinò L, Eberhardt WEE, Poddubskaya E, et al. Nivolumab versus docetaxel in advanced squamous-cell non–small-cell lung cancer. N Engl J Med (2015) 373(2):123–35. doi:10.1056/NEJMoa1504627
24. Motzer RJ, Escudier B, McDermott DF, George S, Hammers HJ, Srinivas S, et al. Nivolumab versus everolimus in advanced renal-cell carcinoma. N Engl J Med (2015) 373(19):1803–13. doi:10.1056/NEJMoa1510665
25. Ansell SM, Lesokhin AM, Borrello I, Halwani A, Scott EC, Gutierrez M, et al. PD-1 Blockade with nivolumab in relapsed or refractory Hodgkin’s lymphoma. N Engl J Med (2015) 372(4):311–9. doi:10.1056/NEJMoa1411087
26. Muro K, Chung HC, Shankaran V, Geva R, Catenacci D, Gupta S, et al. Pembrolizumab for patients with PD-L1-positive advanced gastric cancer (KEYNOTE-012): a multicentre, open-label, phase 1b trial. Lancet Oncol (2016) 17(6):717–26. doi:10.1016/S1470-2045(16)00175-3
27. Rosenberg JE, Hoffman-Censits J, Powles T, van der Heijden MS, Balar AV, Necchi A, et al. Atezolizumab in patients with locally advanced and metastatic urothelial carcinoma who have progressed following treatment with platinum-based chemotherapy: a single-arm, multicentre, phase 2 trial. Lancet (2016) 387(10031):1909–20. doi:10.1016/S0140-6736(16)00561-4
28. Zitvogel L, Galluzzi L, Smyth MJ, Kroemer G. Mechanism of action of conventional and targeted anticancer therapies: reinstating immunosurveillance. Immunity (2013) 39(1):74–88. doi:10.1016/j.immuni.2013.06.014
29. Blank CU, Haanen JB, Ribas A, Schumacher TN. The “cancer immunogram”. Science (2016) 352(6286):658–60. doi:10.1126/science.aaf2834
30. Bezu L, Gomes-de-Silva LC, Dewitte H, Breckpot K, Fucikova J, Spisek R, et al. Combinatorial strategies for the induction of immunogenic cell death. Front Immunol (2015) 6:187. doi:10.3389/fimmu.2015.00187
31. Vandenabeele P, Vandecasteele K, Bachert C, Krysko O, Krysko DV. Immunogenic Apoptotic Cell Death and Anticancer Immunity. New York: Springer International Publishing (2016). p. 133–49.
32. Martin K, Schreiner J, Zippelius A. Modulation of APC function and anti-tumor immunity by anti-cancer drugs. Front Immunol (2015) 6:501. doi:10.3389/fimmu.2015.00501
33. Pfirschke C, Engblom C, Rickelt S, Cortez-Retamozo V, Garris C, Pucci F, et al. Immunogenic chemotherapy sensitizes tumors to checkpoint blockade therapy. Immunity (2016) 44(2):343–54. doi:10.1016/j.immuni.2015.11.024
34. van der Sluis TC, van der Burg SH, Melief CJ. Synergy between chemotherapy and cancer vaccination. Aging (Albany NY) (2015) 7(6):340–1. doi:10.18632/aging.100752
35. van der Sluis TC, van Duikeren S, Huppelschoten S, Jordanova ES, Beyranvand Nejad E, Sloots A, et al. Vaccine-induced tumor necrosis factor-producing T cells synergize with cisplatin to promote tumor cell death. Clin Cancer Res (2015) 21(4):781–94. doi:10.1158/1078-0432.CCR-14-2142
36. Hersh EM, O’Day SJ, Powderly J, Khan KD, Pavlick AC, Cranmer LD, et al. A phase II multicenter study of ipilimumab with or without dacarbazine in chemotherapy-naïve patients with advanced melanoma. Invest New Drugs (2011) 29(3):489–98. doi:10.1007/s10637-009-9376-8
37. Robert C, Thomas L, Bondarenko I, O’Day S, Weber J, Garbe C, et al. Ipilimumab plus dacarbazine for previously untreated metastatic melanoma. N Engl J Med (2011) 364(26):2517–26. doi:10.1056/NEJMoa1104621
38. Lynch TJ, Bondarenko I, Luft A, Serwatowski P, Barlesi F, Chacko R, et al. Ipilimumab in combination with paclitaxel and carboplatin as first-line treatment in stage IIIB/IV non-small-cell lung cancer: results from a randomized, double-blind, multicenter phase II study. J Clin Oncol (2012) 30(17):2046–54. doi:10.1200/JCO.2011.38.4032
39. Reck M, Bondarenko I, Luft A, Serwatowski P, Barlesi F, Chacko R, et al. Ipilimumab in combination with paclitaxel and carboplatin as first-line therapy in extensive-disease-small-cell lung cancer: results from a randomized, double-blind, multicenter phase 2 trial. Ann Oncol (2012) 24(1):75–83. doi:10.1093/annonc/mds213
40. Michaud M, Martins I, Sukkurwala AQ, Adjemian S, Ma Y, Pellegatti P, et al. Autophagy-dependent anticancer immune responses induced by chemotherapeutic agents in mice. Science (2011) 334(6062):1573–7. doi:10.1126/science.1208347
41. Michaud M, Sukkurwala AQ, Martins I, Shen S, Zitvogel L, Kroemer G. Subversion of the chemotherapy-induced anticancer immune response by the ecto-ATPase CD39. Oncoimmunology (2012) 1(3):393–5. doi:10.4161/onci.19070
42. Frederick DT, Piris A, Cogdill AP, Cooper ZA, Lezcano C, Ferrone CR, et al. BRAF inhibition is associated with enhanced melanoma antigen expression and a more favorable tumor microenvironment in patients with metastatic melanoma. Clin Cancer Res (2013) 19(5):1225–31. doi:10.1158/1078-0432.CCR-12-1630
43. Ribas A, Hodi FS, Callahan M, Konto C, Wolchok J. Hepatotoxicity with combination of vemurafenib and ipilimumab. N Engl J Med (2013) 368(14):1365–6. doi:10.1056/NEJMc1302338
44. Puzanov I, Callahan M, Linette G, Patel S, Luke J, Sosman J. Phase I study of the BRAF inhibitor dabrafenib (D) with or without the MEK inhibitor trametinib (T) in combination with ipilimumab (Ipi) for V600E/K mutation-positive unresectable or metastatic melanoma. J Clin Oncol (2014) 32(5s):suppl; abstr 2511.
45. Minor DR, Puzanov I, Callahan MK, Hug BA, Hoos A. Severe gastrointestinal toxicity with administration of trametinib in combination with dabrafenib and ipilimumab. Pigment Cell Melanoma Res (2015) 28(5):611–2. doi:10.1111/pcmr.12383
46. Cooper ZA, Juneja VR, Sage PT, Frederick DT, Piris A, Mitra D, et al. Response to BRAF inhibition in melanoma is enhanced when combined with immune checkpoint blockade. Cancer Immunol Res (2014) 2(7):643–54. doi:10.1158/2326-6066.CIR-13-0215
47. Hu-Lieskovan S, Robert L, Homet Moreno B, Ribas A. Combining targeted therapy with immunotherapy in BRAF-mutant melanoma: promise and challenges. J Clin Oncol (2014) 32(21):2248–54. doi:10.1200/JCO.2013.52.1377
48. Vella LJ, Pasam A, Dimopoulos N, Andrews M, Knights A, Puaux A-L, et al. MEK inhibition, alone or in combination with BRAF inhibition, affects multiple functions of isolated normal human lymphocytes and dendritic cells. Cancer Immunol Res (2014) 2(4):351–60. doi:10.1158/2326-6066.CIR-13-0181
49. Liu L, Mayes PA, Eastman S, Shi H, Yadavilli S, Zhang T, et al. The BRAF and MEK inhibitors dabrafenib and trametinib: effects on immune function and in combination with immunomodulatory antibodies targeting PD-1, PD-L1, and CTLA-4. Clin Cancer Res (2015) 21(7):1639–51. doi:10.1158/1078-0432.CCR-14-2339
50. Ribas A, Butler M, Lutzky J, Lawrence D, Robert C, Miller W, et al. Phase I study combining anti-PD-L1 (MEDI4736) with BRAF (dabrafenib) and/or MEK (trametinib) inhibitors in advanced melanoma. J Clin Oncol (2015) 33:suppl; abstr 3003.
51. Reits EA, Hodge JW, Herberts CA, Groothuis TA, Chakraborty M, Wansley EK, et al. Radiation modulates the peptide repertoire, enhances MHC class I expression, and induces successful antitumor immunotherapy. J Exp Med (2006) 203(5):1259–71. doi:10.1084/jem.20052494
52. Sharma A, Bode B, Wenger RH, Lehmann K, Sartori AA, Moch H, et al. γ-Radiation promotes immunological recognition of cancer cells through increased expression of cancer-testis antigens in vitro and in vivo. PLoS One (2011) 6(11):e28217. doi:10.1371/journal.pone.0028217
53. Vanpouille-Box C, Diamond JM, Pilones KA, Zavadil J, Babb JS, Formenti SC, et al. TGFβ is a master regulator of radiation therapy-induced antitumor immunity. Cancer Res (2015) 75(11):2232–42. doi:10.1158/0008-5472.CAN-14-3511
54. Lugade AA, Moran JP, Gerber SA, Rose RC, Frelinger JG, Lord EM. Local radiation therapy of B16 melanoma tumors increases the generation of tumor antigen-specific effector cells that traffic to the tumor. J Immunol (2005) 174(12):7516–23. doi:10.4049/jimmunol.174.12.7516
55. Lee Y, Auh SL, Wang YY, Burnette B, Wang YY, Meng Y, et al. Therapeutic effects of ablative radiation on local tumor require CD8+ T cells: changing strategies for cancer treatment. Blood (2009) 114(3):589–95. doi:10.1182/blood-2009-02-206870
56. Vatner RE, Cooper BT, Vanpouille-Box C, Demaria S, Formenti SC. Combinations of immunotherapy and radiation in cancer therapy. Front Oncol (2014) 4:325. doi:10.3389/fonc.2014.00325
57. Apetoh L, Ghiringhelli F, Tesniere A, Criollo A, Ortiz C, Lidereau R, et al. The interaction between HMGB1 and TLR4 dictates the outcome of anticancer chemotherapy and radiotherapy. Immunol Rev (2007) 220:47–59. doi:10.1111/j.1600-065X.2007.00573.x
58. Lomax ME, Folkes LK, O’Neill P. Biological consequences of radiation-induced DNA damage: relevance to radiotherapy. Clin Oncol (R Coll Radiol) (2013) 25(10):578–85. doi:10.1016/j.clon.2013.06.007
59. Demaria S, Golden EB, Formenti SC. Role of local radiation therapy in cancer immunotherapy. JAMA Oncol (2015) 1(9):1325–32. doi:10.1001/jamaoncol.2015.2756
60. Abuodeh Y, Venkat P, Kim S. Systematic review of case reports on the abscopal effect. Curr Probl Cancer (2016) 40(1):25–37. doi:10.1016/j.currproblcancer.2015.10.001
61. Demaria S, Kawashima N, Yang AM, Devitt ML, Babb JS, Allison JP, et al. Immune-mediated inhibition of metastases after treatment with local radiation and CTLA-4 blockade in a mouse model of breast cancer. Clin Cancer Res (2005) 11(2 Pt 1):728–34.
62. Ruocco MG, Pilones KA, Kawashima N, Cammer M, Huang J, Babb JS, et al. Suppressing T cell motility induced by anti-CTLA-4 monotherapy improves antitumor effects. J Clin Invest (2012) 122(10):3718–30. doi:10.1172/JCI61931
63. Sharabi AB, Nirschl CJ, Kochel CM, Nirschl TR, Francica BJ, Velarde E, et al. Stereotactic radiation therapy augments antigen-specific PD-1-mediated antitumor immune responses via cross-presentation of tumor antigen. Cancer Immunol Res (2015) 3(4):345–55. doi:10.1158/2326-6066.CIR-14-0196
64. Zeng J, See AP, Phallen J, Jackson CM, Belcaid Z, Ruzevick J, et al. Anti-PD-1 blockade and stereotactic radiation produce long-term survival in mice with intracranial gliomas. Int J Radiat Oncol Biol Phys (2013) 86(2):343–9. doi:10.1016/j.ijrobp.2012.12.025
65. Postow MA, Callahan MK, Barker CA, Yamada Y, Yuan J, Kitano S, et al. Immunologic correlates of the abscopal effect in a patient with melanoma. N Engl J Med (2012) 366(10):925–31. doi:10.1056/NEJMoa1112824
66. Golden EB, Demaria S, Schiff PB, Chachoua A, Formenti SC. An abscopal response to radiation and ipilimumab in a patient with metastatic non-small cell lung cancer. Cancer Immunol Res (2013) 1(6):365–72. doi:10.1158/2326-6066.CIR-13-0115
67. Slovin SF, Higano CS, Hamid O, Tejwani S, Harzstark A, Alumkal JJ, et al. Ipilimumab alone or in combination with radiotherapy in metastatic castration-resistant prostate cancer: results from an open-label, multicenter phase I/II study. Ann Oncol (2013) 24(7):1813–21. doi:10.1093/annonc/mdt107
68. Kwon ED, Drake CG, Scher HI, Fizazi K, Bossi A, van den Eertwegh AJ, et al. Ipilimumab versus placebo after radiotherapy in patients with metastatic castration-resistant prostate cancer that had progressed after docetaxel chemotherapy (CA184-043): a multicentre, randomised, double-blind, phase 3 trial. Lancet Oncol (2014) 15(7):700–12. doi:10.1016/S1470-2045(14)70189-5
69. Victor CT-S, Rech AJ, Maity A, Rengan R, Pauken KE, Stelekati E, et al. Radiation and dual checkpoint blockade activate non-redundant immune mechanisms in cancer. Nature (2015) 520(7547):373–7. doi:10.1038/nature14292
70. Teng F, Kong L, Meng X, Yang J, Yu J. Radiotherapy combined with immune checkpoint blockade immunotherapy: achievements and challenges. Cancer Lett (2015) 365(1):23–9. doi:10.1016/j.canlet.2015.05.012
71. Demaria S, Pilones KA, Vanpouille-Box C, Golden EB, Formenti SC. The optimal partnership of radiation and immunotherapy: from preclinical studies to clinical translation. Radiat Res (2014) 182(2):170–81. doi:10.1667/RR13500.1
72. Demaria S, Formenti SC. Radiation as an immunological adjuvant: current evidence on dose and fractionation. Front Oncol (2012) 2:153. doi:10.3389/fonc.2012.00153
73. Vanpouille-Box C, Pilones KA, Wennerberg E, Formenti SC, Demaria S. In situ vaccination by radiotherapy to improve responses to anti-CTLA-4 treatment. Vaccine (2015) 33(51):7415–22. doi:10.1016/j.vaccine.2015.05.105
74. Rosenberg SA, Sherry RM, Morton KE, Scharfman WJ, Yang JC, Topalian SL, et al. Tumor progression can occur despite the induction of very high levels of self/tumor antigen-specific CD8+ T cells in patients with melanoma. J Immunol (2005) 175(9):6169–76. doi:10.4049/jimmunol.175.9.6169
75. Gibney GT, Kudchadkar RR, DeConti RC, Thebeau MS, Czupryn MP, Tetteh L, et al. Safety, correlative markers, and clinical results of adjuvant nivolumab in combination with vaccine in resected high-risk metastatic melanoma. Clin Cancer Res (2015) 21(4):712–20. doi:10.1158/1078-0432.CCR-14-2468
76. Ribas A, Comin-Anduix B, Chmielowski B, Jalil J, de la Rocha P, McCannel TA, et al. Dendritic cell vaccination combined with CTLA4 blockade in patients with metastatic melanoma. Clin Cancer Res (2009) 15(19):6267–76. doi:10.1158/1078-0432.CCR-09-1254
77. Geary SM, Lemke CD, Lubaroff DM, Salem AK. Proposed mechanisms of action for prostate cancer vaccines. Nat Rev Urol (2013) 10(3):149–60. doi:10.1038/nrurol.2013.8
78. Kaufman HL, Ruby CE, Hughes T, Slingluff CL. Current status of granulocyte-macrophage colony-stimulating factor in the immunotherapy of melanoma. J Immunother cancer (2014) 2:11. doi:10.1186/2051-1426-2-11
79. van Elsas A, Hurwitz AA, Allison JP. Combination immunotherapy of B16 melanoma using anti-cytotoxic T lymphocyte-associated antigen 4 (CTLA-4) and granulocyte/macrophage colony-stimulating factor (GM-CSF)-producing vaccines induces rejection of subcutaneous and metastatic tumors accompanied. J Exp Med (1999) 190(3):355–66. doi:10.1084/jem.190.3.355
80. Quezada SA, Peggs KS, Curran MA, Allison JP. CTLA4 blockade and GM-CSF combination immunotherapy alters the intratumor balance of effector and regulatory T cells. J Clin Invest (2006) 116(7):1935–45. doi:10.1172/JCI27745
81. Li B, VanRoey M, Wang C, Chen TT, Korman A, Jooss K. Anti-programmed death-1 synergizes with granulocyte macrophage colony-stimulating factor – secreting tumor cell immunotherapy providing therapeutic benefit to mice with established tumors. Clin Cancer Res (2009) 15(5):1623–34. doi:10.1158/1078-0432.CCR-08-1825
82. Soares KC, Rucki AA, Wu AA, Olino K, Xiao Q, Chai Y, et al. PD-1/PD-L1 blockade together with vaccine therapy facilitates effector T-cell infiltration into pancreatic tumors. J Immunother (2015) 38(1):1–11. doi:10.1097/CJI.0000000000000062
83. Duraiswamy J, Kaluza KM, Freeman GJ, Coukos G. Dual blockade of PD-1 and CTLA-4 combined with tumor vaccine effectively restores T-cell rejection function in tumors. Cancer Res (2013) 73(12):3591–603. doi:10.1158/0008-5472.CAN-12-4100
84. van den Eertwegh AJM, Versluis J, van den Berg HP, Santegoets SJAM, van Moorselaar RJA, van der Sluis TM, et al. Combined immunotherapy with granulocyte-macrophage colony-stimulating factor-transduced allogeneic prostate cancer cells and ipilimumab in patients with metastatic castration-resistant prostate cancer: a phase 1 dose-escalation trial. Lancet Oncol (2012) 13(5):509–17. doi:10.1016/S1470-2045(12)70007-4
85. Hodi FS, Lee S, McDermott DF, Rao UN, Butterfield LH, Tarhini AA, et al. Ipilimumab plus sargramostim vs ipilimumab alone for treatment of metastatic melanoma: a randomized clinical trial. JAMA (2014) 312(17):1744–53. doi:10.1001/jama.2014.13943
86. Johnson DB, Puzanov I, Kelley MC. Talimogene laherparepvec (T-VEC) for the treatment of advanced melanoma. Immunotherapy (2015) 7(6):611–9. doi:10.2217/imt.15.35
87. Long GV, Dummer R, Ribas A, Puzanov I, Michielin O, VanderWalde A, et al. A phase I/III, multicenter, open-label trial of talimogene laherparepvec (T-VEC) in combination with pembrolizumab for the treatment of unresected, stage IIIb-IV melanoma (MASTERKEY-265). J Immunother Cancer (2015) 3(Suppl 2):181. doi:10.1186/2051-1426-3-S2-P181
88. Long GV, Dummer R, Ribas A, Puzanov I, Walde A. Efficacy analysis of MASTERKEY-265 phase 1b study of talimogene laherparepvec (T-VEC) and pembrolizumab (pembro) for unresectable stage IIIB-IV melanoma. J Clin Oncol (2016) 34:suppl; abstr 9568.
89. Bajor DL, Mick R, Riese MJ, Richman LP, Xu X, Torigian DA, et al. Abstract CT137: combination of agonistic CD40 monoclonal antibody CP-870,893 and anti-CTLA-4 antibody tremelimumab in patients with metastatic melanoma. Cancer Res (2015) 75(15 Suppl):CT137. doi:10.1158/1538-7445.AM2015-CT137
90. Zippelius A, Schreiner J, Herzig P, Müller P. Induced PD-L1 expression mediates acquired resistance to agonistic anti-CD40 treatment. Cancer Immunol Res (2015) 3(3):236–44. doi:10.1158/2326-6066.CIR-14-0226
91. Liu X, Pu Y, Cron K, Deng L, Kline J, Frazier WA, et al. CD47 blockade triggers T cell-mediated destruction of immunogenic tumors. Nat Med (2015) 21(10):1209–15. doi:10.1038/nm.3931
92. Curran MA, Montalvo W, Yagita H, Allison JP. PD-1 and CTLA-4 combination blockade expands infiltrating T cells and reduces regulatory T and myeloid cells within B16 melanoma tumors. Proc Natl Acad Sci U S A (2010) 107(9):4275–80. doi:10.1073/pnas.0915174107
93. Lussier DM, Johnson JL, Hingorani P, Blattman JN. Combination immunotherapy with α-CTLA-4 and α-PD-L1 antibody blockade prevents immune escape and leads to complete control of metastatic osteosarcoma. J Immunother Cancer (2015) 3(1):21. doi:10.1186/s40425-015-0067-z
94. Selby M, Engelhardt J, Lu L, Quigley M, Wang C, Chen B, et al. Antitumor activity of concurrent blockade of immune checkpoint molecules CTLA-4 and PD-1 in preclinical models. J Clin Oncol (2013) 31:suppl; abstr 3061.
95. Postow MA, Chesney J, Pavlick AC, Robert C, Grossmann K, McDermott D, et al. Nivolumab and ipilimumab versus ipilimumab in untreated melanoma. N Engl J Med (2015) 372(21):2006–17. doi:10.1056/NEJMoa1414428
96. Larkin J, Chiarion-Sileni V, Gonzalez R, Grob JJ, Cowey CL, Lao CD, et al. Combined nivolumab and ipilimumab or monotherapy in untreated melanoma. N Engl J Med (2015) 373(1):23–34. doi:10.1056/NEJMoa1504030
97. Grosso JF, Goldberg MV, Getnet D, Bruno TC, Yen H-R, Pyle KJ, et al. Functionally distinct LAG-3 and PD-1 subsets on activated and chronically stimulated CD8 T cells. J Immunol (2009) 182(11):6659–69. doi:10.4049/jimmunol.0804211
98. Matsuzaki J, Gnjatic S, Mhawech-Fauceglia P, Beck A, Miller A, Tsuji T, et al. Tumor-infiltrating NY-ESO-1-specific CD8+ T cells are negatively regulated by LAG-3 and PD-1 in human ovarian cancer. Proc Natl Acad Sci U S A (2010) 107(17):7875–80. doi:10.1073/pnas.1003345107
99. Jones RB, Ndhlovu LC, Barbour JD, Sheth PM, Jha AR, Long BR, et al. Tim-3 expression defines a novel population of dysfunctional T cells with highly elevated frequencies in progressive HIV-1 infection. J Exp Med (2008) 205(12):2763–79. doi:10.1084/jem.20081398
100. Johnston RJ, Comps-Agrar L, Hackney J, Yu X, Huseni M, Yang Y, et al. The immunoreceptor TIGIT regulates antitumor and antiviral CD8+ T cell effector function. Cancer Cell (2014) 26(6):923–37. doi:10.1016/j.ccell.2014.10.018
101. Woo S-R, Turnis ME, Goldberg MV, Bankoti J, Selby M, Nirschl CJ, et al. Immune inhibitory molecules LAG-3 and PD-1 synergistically regulate T-cell function to promote tumoral immune escape. Cancer Res (2012) 72(4):917–27. doi:10.1158/0008-5472.CAN-11-1620
102. Holmgaard RB, Zamarin D, Munn DH, Wolchok JD, Allison JP. Indoleamine 2,3-dioxygenase is a critical resistance mechanism in antitumor T cell immunotherapy targeting CTLA-4. J Exp Med (2013) 210(7):1389–402. doi:10.1084/jem.20130066
103. Spranger S, Koblish HK, Horton B, Scherle PA, Newton R, Gajewski TF. Mechanism of tumor rejection with doublets of CTLA-4, PD-1/PD-L1, or IDO blockade involves restored IL-2 production and proliferation of CD8(+) T cells directly within the tumor microenvironment. J Immunother cancer (2014) 2:3. doi:10.1186/2051-1426-2-3
104. Sakuishi K, Apetoh L, Sullivan JM, Blazar BR, Kuchroo VK, Anderson AC. Targeting Tim-3 and PD-1 pathways to reverse T cell exhaustion and restore anti-tumor immunity. J Exp Med (2010) 207(10):2187–94. doi:10.1084/jem.20100643
105. Kim JE, Patel MA, Mangraviti A, Velarde E, Theodros D, Mathios D, et al. The combination of anti-TIM-3 and anti-PD-1 checkpoint inhibitors with focused radiation resulted in a synergistic antitumor immune response in a preclinical glioma model. Neurosurgery (2015) 62(Suppl 1):212. doi:10.1227/01.neu.0000467105.60300.04
106. Liu J, Yuan Y, Chen W, Putra J, Suriawinata AA, Schenk AD, et al. Immune-checkpoint proteins VISTA and PD-1 nonredundantly regulate murine T-cell responses. Proc Natl Acad Sci U S A (2015) 112(21):6682–7. doi:10.1073/pnas.1420370112
107. Wainwright DA, Chang AL, Dey M, Balyasnikova IV, Kim CK, Tobias A, et al. Durable therapeutic efficacy utilizing combinatorial blockade against IDO, CTLA-4, and PD-L1 in mice with brain tumors. Clin Cancer Res (2014) 20(20):5290–301. doi:10.1158/1078-0432.CCR-14-0514
108. Wang L, Rubinstein R, Lines JL, Wasiuk A, Ahonen C, Guo Y, et al. VISTA, a novel mouse Ig superfamily ligand that negatively regulates T cell responses. J Exp Med (2011) 208(3):577–92. doi:10.1084/jem.20100619
109. Flies DB, Wang S, Xu H, Chen L. Cutting edge: a monoclonal antibody specific for the programmed death-1 homolog prevents graft-versus-host disease in mouse models. J Immunol (2011) 187(4):1537–41. doi:10.4049/jimmunol.1100660
110. Lines JL, Sempere LF, Broughton T, Wang L, Noelle R. VISTA is a novel broad-spectrum negative checkpoint regulator for cancer immunotherapy. Cancer Immunol Res (2014) 2(6):510–7. doi:10.1158/2326-6066.CIR-14-0072
111. Benson DM, Caligiuri MA. Killer immunoglobulin-like receptors and tumor immunity. Cancer Immunol Res (2014) 2(2):99–104. doi:10.1158/2326-6066.CIR-13-0219
112. Guo Z, Wang X, Cheng D, Xia Z, Luan M, Zhang S. PD-1 blockade and OX40 triggering synergistically protects against tumor growth in a murine model of ovarian cancer. PLoS One (2014) 9(2):e89350. doi:10.1371/journal.pone.0089350
113. Redmond WL, Linch SN, Kasiewicz MJ. Combined targeting of costimulatory (OX40) and coinhibitory (CTLA-4) pathways elicits potent effector T cells capable of driving robust antitumor immunity. Cancer Immunol Res (2014) 2(2):142–53. doi:10.1158/2326-6066.CIR-13-0031-T
114. Lu L, Xu X, Zhang B, Zhang R, Ji H, Wang X. Combined PD-1 blockade and GITR triggering induce a potent antitumor immunity in murine cancer models and synergizes with chemotherapeutic drugs. J Transl Med (2014) 12(1):36. doi:10.1186/1479-5876-12-36
115. Kocak E, Lute K, Chang X, May KF, Exten KR, Zhang H, et al. Combination therapy with anti-CTL antigen-4 and anti-4-1BB antibodies enhances cancer immunity and reduces autoimmunity. Cancer Res (2006) 66(14):7276–84. doi:10.1158/0008-5472.CAN-05-2128
116. Belcaid Z, Phallen JA, Zeng J, See AP, Mathios D, Gottschalk C, et al. Focal radiation therapy combined with 4-1BB activation and CTLA-4 blockade yields long-term survival and a protective antigen-specific memory response in a murine glioma model. PLoS One (2014) 9(7):e101764. doi:10.1371/journal.pone.0101764
117. Dai M, Wei H, Yip YY, Feng Q, He K, Popov V, et al. Long-lasting complete regression of established mouse tumors by counteracting Th2 inflammation. J Immunother (2013) 36(4):248–57. doi:10.1097/CJI.0b013e3182943549
118. Jensen BAH, Pedersen SR, Christensen JP, Thomsen AR. The availability of a functional tumor targeting T-cell repertoire determines the anti-tumor efficiency of combination therapy with anti-CTLA-4 and anti-4-1BB antibodies. PLoS One (2013) 8(6):e66081. doi:10.1371/journal.pone.0066081
119. Ahrends T, Babala N, Xiao Y, Yagita H, van Eenennaam H, Borst J. CD27 agonism plus PD-1 blockade recapitulates CD4+ T cell help in therapeutic anti-cancer vaccination. Cancer Res (2016) 76(10):2921–31. doi:10.1158/0008-5472.CAN-15-3130
120. Chen S, Lee L-F, Fisher TS, Jessen B, Elliott M, Evering W, et al. Combination of 4-1BB agonist and PD-1 antagonist promotes antitumor effector/memory CD8 T cells in a poorly immunogenic tumor model. Cancer Immunol Res (2014) 3(2):149–60. doi:10.1158/2326-6066.CIR-14-0118
121. Shindo Y, Yoshimura K, Kuramasu A, Watanabe Y, Ito H, Kondo T, et al. Combination immunotherapy with 4-1BB activation and PD-1 blockade enhances antitumor efficacy in a mouse model of subcutaneous tumor. Anticancer Res (2015) 35(1):129–36.
122. Hirano F, Kaneko K, Tamura H, Dong H, Wang S, Ichikawa M, et al. Blockade of B7-H1 and PD-1 by monoclonal antibodies potentiates cancer therapeutic immunity. Cancer Res (2005) 65(3):1089–96.
123. Ascierto PA, Simeone E, Sznol M, Fu Y-X, Melero I. Clinical experiences with anti-CD137 and anti-PD1 therapeutic antibodies. Semin Oncol (2010) 37(5):508–16. doi:10.1053/j.seminoncol.2010.09.008
124. Bartkowiak T, Curran MA. 4-1BB agonists: multi-potent potentiators of tumor immunity. Front Oncol (2015) 5:117. doi:10.3389/fonc.2015.00117
125. van de Ven K, Borst J. Targeting the T-cell co-stimulatory CD27/CD70 pathway in cancer immunotherapy: rationale and potential. Immunotherapy (2015) 7(6):655–67. doi:10.2217/imt.15.32
126. Jung H. Abstract A90: combination therapy of chemokine receptor inhibition plus PDL-1 blockade potentiates anti-tumor effects in a murine model of breast cancer. Mol Cancer Ther (2015) 14(12 Suppl 2):A90–90. doi:10.1158/1535-7163.TARG-15-A90
127. Highfill SL, Cui Y, Giles AJ, Smith JP, Zhang H, Morse E, et al. Disruption of CXCR2-mediated MDSC tumor trafficking enhances anti-PD1 efficacy. Sci Transl Med (2014) 6(237):237ra67. doi:10.1126/scitranslmed.3007974
128. Sugiyama D, Nishikawa H, Maeda Y, Nishioka M, Tanemura A, Katayama I, et al. Anti-CCR4 mAb selectively depletes effector-type FoxP3+CD4+ regulatory T cells, evoking antitumor immune responses in humans. Proc Natl Acad Sci U S A (2013) 110(44):17945–50. doi:10.1073/pnas.1316796110
129. Chang D-K, Sui J, Geng S, Muvaffak A, Bai M, Fuhlbrigge RC, et al. Humanization of an anti-CCR4 antibody that kills cutaneous T-cell lymphoma cells and abrogates suppression by T-regulatory cells. Mol Cancer Ther (2012) 11(11):2451–61. doi:10.1158/1535-7163.MCT-12-0278
130. Dirkx AEM, oude Egbrink MG, Castermans K, van der Schaft DW, Thijssen VL, Dings RP, et al. Anti-angiogenesis therapy can overcome endothelial cell anergy and promote leukocyte-endothelium interactions and infiltration in tumors. FASEB J (2006) 20(6):621–30. doi:10.1096/fj.05-4493com
131. Voron T, Colussi O, Marcheteau E, Pernot S, Nizard M, Pointet A-L, et al. VEGF-A modulates expression of inhibitory checkpoints on CD8+ T cells in tumors. J Exp Med (2015) 212(2):139–48. doi:10.1084/jem.20140559
132. Ohm JE, Carbone DP. VEGF as a mediator of tumor-associated immunodeficiency. Immunol Res (2001) 23(2–3):263–72. doi:10.1385/IR:23:2-3:263
133. Terme M, Pernot S, Marcheteau E, Sandoval F, Benhamouda N, Colussi O, et al. VEGFA-VEGFR pathway blockade inhibits tumor-induced regulatory T-cell proliferation in colorectal cancer. Cancer Res (2013) 73(2):539–49. doi:10.1158/0008-5472.CAN-12-2325
134. Yuan J, Zhou J, Dong Z, Tandon S, Kuk D, Panageas KS, et al. Pretreatment serum VEGF is associated with clinical response and overall survival in advanced melanoma patients treated with ipilimumab. Cancer Immunol Res (2014) 2(2):127–32. doi:10.1158/2326-6066.CIR-13-0163
135. Hodi FS, Lawrence D, Lezcano C, Wu X, Zhou J, Sasada T, et al. Bevacizumab plus ipilimumab in patients with metastatic melanoma. Cancer Immunol Res (2014) 2(7):632–42. doi:10.1158/2326-6066.CIR-14-0053
136. Rini BI, Stein M, Shannon P, Eddy S, Tyler A, Stephenson JJ, et al. Phase 1 dose-escalation trial of tremelimumab plus sunitinib in patients with metastatic renal cell carcinoma. Cancer (2011) 117(4):758–67. doi:10.1002/cncr.25639
137. Amin A, Plimack E, Infante J. Nivolumab (anti-PD-1; BMS-936558, ONO-4538) in combination with sunitinib or pazopanib in patients (pts) with metastatic renal cell carcinoma (mRCC). J Clin Oncol (2014) 32(5s):suppl; abstr 5010.
138. Ott PA, Hodi FS, Buchbinder EI. Inhibition of immune checkpoints and vascular endothelial growth factor as combination therapy for metastatic melanoma: an overview of rationale, preclinical evidence, and initial clinical data. Front Oncol (2015) 5:202. doi:10.3389/fonc.2015.00202
139. Lu H. TLR agonists for cancer immunotherapy: tipping the balance between the immune stimulatory and inhibitory effects. Front Immunol (2014) 5:83. doi:10.3389/fimmu.2014.00083
140. Dowling JK, Mansell A. Toll-like receptors: the swiss army knife of immunity and vaccine development. Clin Transl Immunology (2016) 5(5):e85. doi:10.1038/cti.2016.22
141. Shirota Y, Shirota H, Klinman DM. Intratumoral injection of CpG oligonucleotides induces the differentiation and reduces the immunosuppressive activity of myeloid-derived suppressor cells. J Immunol (2012) 188(4):1592–9. doi:10.4049/jimmunol.1101304
142. Karpf AR, Jones DA. Reactivating the expression of methylation silenced genes in human cancer. Oncogene (2002) 21(35):5496–503. doi:10.1038/sj.onc.1205602
143. De Smet C, De Backer O, Faraoni I, Lurquin C, Brasseur F, Boon T. The activation of human gene MAGE-1 in tumor cells is correlated with genome-wide demethylation. Proc Natl Acad Sci U S A (1996) 93(14):7149–53. doi:10.1073/pnas.93.14.7149
144. Héninger E, Krueger TEG, Lang JM. Augmenting antitumor immune responses with epigenetic modifying agents. Front Immunol (2015) 6:29. doi:10.3389/fimmu.2015.00029
145. Wang L-X, Mei Z-Y, Zhou J-H, Yao Y-S, Li Y-H, Xu Y-H, et al. Low dose decitabine treatment induces CD80 expression in cancer cells and stimulates tumor specific cytotoxic T lymphocyte responses. PLoS One (2013) 8(5):e62924. doi:10.1371/journal.pone.0062924
146. Covre A, Coral S, Nicolay H, Parisi G, Fazio C, Colizzi F, et al. Antitumor activity of epigenetic immunomodulation combined with CTLA-4 blockade in syngeneic mouse models. Oncoimmunology (2015) 4(8):e1019978. doi:10.1080/2162402X.2015.1019978
147. Rosenberg SA, Yang JC, Sherry RM, Kammula US, Hughes MS, Phan GQ, et al. Durable complete responses in heavily pretreated patients with metastatic melanoma using t-cell transfer immunotherapy. Clin Cancer Res (2011) 17(13):4550–7. doi:10.1158/1078-0432.CCR-11-0116
148. Kalos M, June CH. Adoptive T cell transfer for cancer immunotherapy in the era of synthetic biology. Immunity (2013) 39(1):49–60. doi:10.1016/j.immuni.2013.07.002
149. Wu R, Forget M-A, Chacon J, Bernatchez C, Haymaker C, Chen JQ, et al. Adoptive T-cell therapy using autologous tumor-infiltrating lymphocytes for metastatic melanoma: current status and future outlook. Cancer J (2012) 18(2):160–75. doi:10.1097/PPO.0b013e31824d4465
150. Peng W, Liu C, Xu C, Lou Y, Chen J, Yang Y, et al. PD-1 blockade enhances T-cell migration to tumors by elevating IFN-γ inducible chemokines. Cancer Res (2012) 72(20):5209–18. doi:10.1158/0008-5472.CAN-12-1187
151. Blake SJP, Ching ALH, Kenna TJ, Galea R, Large J, Yagita H, et al. Blockade of PD-1/PD-L1 promotes adoptive T-cell immunotherapy in a tolerogenic environment. PLoS One (2015) 10(3):e0119483. doi:10.1371/journal.pone.0119483
152. Mahvi DA, Meyers JV, Tatar AJ, Contreras A, Suresh M, Leverson GE, et al. Ctla-4 blockade plus adoptive T-cell transfer promotes optimal melanoma immunity in mice. J Immunother (2015) 38(2):54–61. doi:10.1097/CJI.0000000000000064
153. John LB, Devaud C, Duong CPM, Yong CS, Beavis PA, Haynes NM, et al. Anti-PD-1 antibody therapy potently enhances the eradication of established tumors by gene-modified t cells. Clin Cancer Res (2013) 19(20):5636–46. doi:10.1158/1078-0432.CCR-13-0458
154. Bjoern J, Donia M, Andersen R, Hadrup S, Lyngaa R, Svane I. Effects of ipilimumab on expanded tumor-infiltrating lymphocytes in patients with stage IV malignant melanoma. J Clin Oncol (2014) 32(5s):suppl; abstr 3020.
155. Zhang H, Conrad DM, Butler JJ, Zhao C, Blay J, Hoskin DW. Adenosine acts through A2 receptors to inhibit IL-2-induced tyrosine phosphorylation of STAT5 in T lymphocytes: role of cyclic adenosine 3’,5’-monophosphate and phosphatases. J Immunol (2004) 173(2):932–44. doi:10.4049/jimmunol.173.2.932
156. Huang S, Apasov S, Koshiba M, Sitkovsky M. Role of A2a extracellular adenosine receptor-mediated signaling in adenosine-mediated inhibition of T-cell activation and expansion. Blood (1997) 90(4):1600–10.
157. Ohta A, Ohta A, Madasu M, Kini R, Subramanian M, Goel N, et al. A2A adenosine receptor may allow expansion of T cells lacking effector functions in extracellular adenosine-rich microenvironments. J Immunol (2009) 183(9):5487–93. doi:10.4049/jimmunol.0901247
158. Mittal D, Sinha D, Barkauskas D, Young A, Kalimutho M, Stannard K, et al. Adenosine 2B receptor expression on cancer cells promotes metastasis. Cancer Res (2016) 76(15):4372–82. doi:10.1158/0008-5472.CAN-16-0544
159. Iannone R, Miele L, Maiolino P, Pinto A, Morello S. Adenosine limits the therapeutic effectiveness of anti-CTLA4 mAb in a mouse melanoma model. Am J Cancer Res (2014) 4(2):172–81.
160. Mittal D, Young A, Stannard K, Yong M, Teng MWL, Allard B, et al. Antimetastatic effects of blocking PD-1 and the adenosine A2A receptor. Cancer Res (2014) 74(14):3652–8. doi:10.1158/0008-5472.CAN-14-0957
161. Beavis PA, Milenkovski N, Henderson MA, John LB, Allard B, Loi S, et al. Adenosine receptor 2A blockade increases the efficacy of Anti-PD-1 through enhanced antitumor T-cell responses. Cancer Immunol Res (2015) 3(5):506–17. doi:10.1158/2326-6066.CIR-14-0211
162. Stagg J, Divisekera U, McLaughlin N, Sharkey J, Pommey S, Denoyer D, et al. Anti-CD73 antibody therapy inhibits breast tumor growth and metastasis. Proc Natl Acad Sci U S A (2010) 107(4):1547–52. doi:10.1073/pnas.0908801107
163. Allard B, Turcotte M, Spring K, Pommey S, Royal I, Stagg J. Anti-CD73 therapy impairs tumor angiogenesis. Int J Cancer (2014) 134(6):1466–73. doi:10.1002/ijc.28456
164. Loi S, Pommey S, Haibe-Kains B, Beavis PA, Darcy PK, Smyth MJ, et al. CD73 promotes anthracycline resistance and poor prognosis in triple negative breast cancer. Proc Natl Acad Sci U S A (2013) 110(27):11091–6. doi:10.1073/pnas.1222251110
165. Turcotte M, Spring K, Pommey S, Chouinard G, Cousineau I, George J, et al. CD73 is associated with poor prognosis in high-grade serous ovarian cancer. Cancer Res (2015) 75(21):4494–503. doi:10.1158/0008-5472.CAN-14-3569
166. Leclerc BG, Charlebois R, Chouinard G, Allard B, Pommey S, Saad F, et al. CD73 expression is an independent prognostic factor in prostate cancer. Clin Cancer Res (2016) 22(1):158–66. doi:10.1158/1078-0432.CCR-15-1181
167. Beavis PA, Slaney CY, Milenkovski N, Henderson MA, Loi S, Stagg J, et al. CD73: a potential biomarker for anti-PD-1 therapy. Oncoimmunology (2015) 4(11):e1046675. doi:10.1080/2162402X.2015.1046675
168. Allard B, Pommey S, Smyth MJ, Stagg J. Targeting CD73 enhances the antitumor activity of anti-PD-1 and anti-CTLA-4 mAbs. Clin Cancer Res (2013) 19(20):5626–35. doi:10.1158/1078-0432.CCR-13-0545
169. Lebrun J-J, Lebrun J-J. The dual role of TGF β in human cancer: from tumor suppression to cancer metastasis. ISRN Mol Biol (2012) 2012:1–28. doi:10.5402/2012/381428
170. Hanks B, Holtzhausen A, Evans K, Heid M, Blobe GC. Combinatorial TGF-β signaling blockade and anti-CTLA-4 antibody immunotherapy in a murine BRAFV600E-PTEN-/-transgenic model of melanoma. J Clin Oncol (2014) 32(5s):suppl; abstr 3011.
171. Munn DH, Mellor AL. IDO and tolerance to tumors. Trends Mol Med (2004) 10(1):15–8. doi:10.1016/j.molmed.2003.11.003
172. Gangadhar TC, Hamid O, Smith DC, Bauer TM, Wasser JS, Luke JJ, et al. Preliminary results from a Phase I/II study of epacadostat (incb024360) in combination with pembrolizumab in patients with selected advanced cancers. J Immunother Cancer (2015) 3(Suppl 2):O7. doi:10.1186/2051-1426-3-S2-O7
173. Regoes RR, Barber DL, Ahmed R, Antia R. Estimation of the rate of killing by cytotoxic T lymphocytes in vivo. Proc Natl Acad Sci U S A (2007) 104(5):1599–603. doi:10.1073/pnas.0508830104
Keywords: cancer immunotherapy, checkpoint blockade, CTLA-4, PD-1
Citation: Swart M, Verbrugge I and Beltman JB (2016) Combination Approaches with Immune-Checkpoint Blockade in Cancer Therapy. Front. Oncol. 6:233. doi: 10.3389/fonc.2016.00233
Received: 20 August 2016; Accepted: 18 October 2016;
Published: 01 November 2016
Edited by:
Sherven Sharma, VA Greater Los Angeles Healthcare System (VHA), USAReviewed by:
John Stagg, Université de Montréal, CanadaAlexandre Corthay, University of Oslo, Norway
Copyright: © 2016 Swart, Verbrugge and Beltman. This is an open-access article distributed under the terms of the Creative Commons Attribution License (CC BY). The use, distribution or reproduction in other forums is permitted, provided the original author(s) or licensor are credited and that the original publication in this journal is cited, in accordance with accepted academic practice. No use, distribution or reproduction is permitted which does not comply with these terms.
*Correspondence: Joost B. Beltman, j.b.beltman@lacdr.leidenuniv.nl